Title Page
Series Page
Copyright
Preface
Chapter 1: Cells and Tissues
Principles of Microscopy
Only Two Types of Cell
Viruses
Origin of Eukaryotic Cells
Cell Specialization in Animals
Stem Cells and Tissue Replacement
The Cell Wall
Chapter 2: Water and Macromolecules
The Chemical Bond: Sharing Electrons
Interactions with Water: Solutions
Biological Macromolecules
Carbohydrates: Candy and Canes
Oxidation and Reduction Involve the Movement of Electrons
Amino Acids, Polypeptides, and Proteins
Lipids
Hydrolysis
Chapter 3: Membranes and Organelles
Basic Properties of Cell Membranes
Organelles Bounded by Double-Membrane Envelopes
Organelles Boundedby Single Membranes
Chapter 4: DNA Structure and The Genetic Code
The Structure of DNA
DNA as the Genetic Material
Packaging of DNA molecules Into Chromosomes
The Genetic Code
Chapter 5: Dna as a Data Storage Medium
DNA Replication
Proteins Open Up the DNA Double Helix During Replication
Biochemistry of DNA Replication
DNA Repair After Replication
Gene Structure and Organization in Eukaryotes
Gene Nomenclature
Chapter 6: Transcription and the Control of Gene Expression
Structure of RNA
RNA Polymerase
Gene Notation
Bacterial RNA Synthesis
Control of Bacterial Gene Expression
Eukaryotic RNA Synthesis
Control of Eukaryotic Gene Expression
Chapter 7: Recombinant DNA and Genetic Engineering
DNA Cloning
Creating the Clone
Uses of DNA Clones
Ethics of DNA Testing for Inherited Disease
Chapter 8: Manufacturing Protein
Attachment of an Amino Acid to Its tRNA
The Ribosome
Bacterial Protein Synthesis
Eukaryotic Protein Synthesis is a Little More Complex
Chapter 9: Protein Structure
Naming Proteins
Polymers of Amino Acids
Other Amino Acids are Found in Nature
The Three-Dimensional Structures of Proteins
Levels of Complexity
Prosthetic Groups
The Primary Structure Contains All The Information Necessary To Specify Higher-Level Structures
Chapter 10: Intracellular Protein Trafficking
Three Modes of Intracellular Protein Transport
Transport to and From The Nucleus
Transport Across Membranes
Vesicular Trafficking Between Intracellular Compartments
Chapter 11: How Proteins Work
How Proteins Bind Other Molecules
Dynamic Protein Structures
Enzymes are Protein Catalysts
Cofactors and Prosthetic Groups
Enzymes can be Regulated
Chapter 12: Energy Trading within the Cell
Cellular Energy Currencies
Energy Currencies Are Interconvertible
Chapter 13: Metabolism
The KREBS Cycle: The Central Switching Yard of Metabolism
From Glucose to Pyruvate: Glycolysis
From Fats to ACETYL-CoA: β Oxidation
Amino Acids as Another Source of Metabolic Energy
Making Glucose: Gluconeogenesis
Making Glycogen: Glycogenesis
Making Fatty Acids, Glycerides and Cholesterol
Synthesis of Amino Acids
Control of Energy Production
Chapter 14: Ions and Voltages
The Potassium Gradient And The Resting Voltage
The Chloride Gradient
General Properties Of Channels
General Properties Of Carriers
Electrical Signaling
Chapter 15: Intracellular Signaling
Calcium
Cyclic Adenosine Monophosphate
Cyclic Guanosine Monophosphate
Multiple Messengers
Biochemical Signaling
Crosstalk—Signaling Pathways Or Signaling Webs?
Chapter 16: Intercellular Communication
Classifying Transmitters And Receptors
Rapid Communication: From Nerve Cells To Their Targets
Paracrine Transmitters And The Control Of Muscle Blood Supply
Chemotaxis
Signaling During Development
Chapter 17: Mechanical Molecules
Microtubules
Microtubule-Based Motility
Microfilaments
Intermediate Filaments
Chapter 18: Cell Cycle and the Control of Cell Number in Eukaryotes
Stages of Mitosis
Meiosis and Fertilization
Control of the Cell Division Cycle
Apoptosis
Chapter 19: The Cell Biology of the Immune System
Cells of the Immune System
B Cells and Antibodies
T Cells
Autoimmune Disease
Chapter 20: Case Study: Cystic Fibrosis
Cystic Fibrosis is a Severe Genetic Disease
The Fundamental Lesion in Cystic Fibrosis Lies in Chloride Transport
Homing in on the CF Gene
Cloning the GENE for CF
The CFTR Gene Codes for a Chloride Ion Channel
Novel Therapies for CF
Diagnostic Tests for CF
The Future
Appendix
Glossary
Answers to Review Questions
Theme: Dimensions in Cell Biology
Theme: Types of Cell
Theme: Some Basic Components of the Eukaryotic Cell
Theme: Types of Organic Chemicals
Theme: Chemical Groups and Bonds
Theme: Acids and Bases
Theme: Membranes
Theme: Organelles in Eukaryotic Cells
Theme: Transport Across Membranes
Theme: Mutations
Theme: Bases and Amino Acids
Theme: Structures Associated with DNA
Theme: Synthesis on a DNA Template
Theme: DNA Replication
Theme: Regions within Eukaryotic Chromosomes
Theme: Codes within the Base Sequence
Theme: The Control of Transcription
Theme: Events that Occur after Transcription in Eukaryotes
Theme: A Mammalian Expression Plasmid
Theme: Choosing an Oligonucleotide for a Specific Task
Theme: Uses of cDNA Clones
Theme: Translation Initiation
Theme: Translation Elongation and Termination
Theme: The Wobble
Theme: Amino Acids
Theme: Terms Used to Describe Proteins
Theme: Specific Binding Partners
Theme: The Three Modes of Intracellular Transport
Theme: Trafficking Processes
Theme: GTPases
Theme: Changing the Preferred Shape of a Protein
Theme: Enzyme Kinetics
Theme: Enzymes
Theme: Cell Spaces and Regions in Energy Trading
Theme: The Electron Transport Chain and ATP Synthase
Theme: Energy Currencies
Theme: Reactions and Pathways
Theme: Pathways and Enzymes
Theme: Metabolism
Theme: Cytosolic and Extracellular Concentrations of Important Ions
Theme: Pathways for Solute Movement Across the Plasma Membrane
Theme: Ion Fluxes in a Nerve Cell
Theme: Processes Downstream of Receptor Tyrosine Kinases
Theme: Proteins Activated by Nucleotides
Theme: Inositol Compounds
Theme: Receptors
Theme: Transmitters
Theme: Synapses
Theme: Cytoskeletal Structures
Theme: Proteins of the Cytoskeleton
Theme: Fueling Movement
Theme: Mitosis
Theme: Checkpoints in the Cell Cycle
Theme: Life and Death
Theme: Immune System Cells
Theme: The Antibody Heavy Chain Locus
Theme: T Cells and Their Interaction with Other Cells
Theme: CFTR Mutations
Theme: The CFTR as a Permeability Pathway
Theme: A Medly of Sentences about CF
Index
Copyright © 2010 by John Wiley & Sons, Inc. All rights reserved.
Published by John Wiley & Sons, Inc., Hoboken, New Jersey.
Published simultaneously in Canada.
No part of this publication may be reproduced, stored in a retrieval system, or transmitted in any form or by any means, electronic, mechanical, photocopying, recording, scanning, or otherwise, except as permitted under Section 107 or 108 of the 1976 United States Copyright Act, without either the prior written permission of the Publisher, or authorization through payment of the appropriate per-copy fee to the Copyright Clearance Center, Inc., 222 Rosewood Drive, Danvers, MA 01923, (978) 750-8400, fax (978) 750-4744. Requests to the Publisher for permission should be addressed to the Permissions Department, John Wiley & Sons, Inc., 111 River Street, Hoboken, NJ 07030, (201) 748-6011, fax (201) 748-6008, or online at http://www.wiley.com/go/permission.
Limit of Liability/Disclaimer of Warranty: While the publisher and author have used their best efforts in preparing this book, they make no representations or warranties with respect to the accuracy or completeness of the contents of this book and specifically disclaim any implied warranties of merchantability or fitness for a particular purpose. No warranty may be created or extended by sales representatives or written sales materials. The advice and strategies contained herein may not be suitable for your situation. You should consult with a professional where appropriate. Neither the publisher nor author shall be liable for any loss of profit or any other commercial damages, including but not limited to special, incidental, consequential, or other damages.
For general information on our other products and services or for technical support, please contact our Customer Care Department within the United States at (800) 762-2974, outside the United States at (317) 572-3993 or fax (317) 572-4002.
Wiley also publishes its books in a variety of electronic formats. Some content that appears in print may not be available in electronic formats. For more information about Wiley products, visit our web site at www.wiley.com.
Library of Congress Cataloging-in-Publication Data:
Cell biology: a short course/ Stephen R. Bolsover ... [et al.]. 3rd ed.
Stephen R. Bolsover Elizabeth A. Shephard Hugh A. White Jeremy S. Hyams
p. cm.
Includes index.
ISBN 978-0-470-52699-6 (pbk.)
1. Cytology. I. Bolsover, Stephen R., 1954-
QH581.2.C425 2011
571.6–dc22
2010040989
Chapter 1
Cells and Tissues
The cell is the basic unit of life. Microorganisms such as bacteria, yeast, and amoebae exist as single cells. By contrast, the adult human is made up of about 30 trillion cells (1 trillion = 1012) which are mostly organized into collectives called tissues. Cells are, with a few notable exceptions, small (Fig. 1.1) with dimensions measured in micrometers (μm, 1μm = 1/1000 mm) and their discovery stemmed from the conviction of a small group of seventeenth-century microscope makers that a new and undiscovered world lay beyond the limits of resolution of the human eye. These pioneers set in motion a science and an industry that continues to the present day.
The first person to observe and record cells was Robert Hooke (1635–1703) who described the cella (open spaces) of plant tissues. But the colossus of this era of discovery was a Dutchman, Anton van Leeuwenhoek (1632–1723), a man with no scientific training but with unrivaled talents as both a microscope maker and as an observer and recorder of the microscopic living world. van Leeuwenhoek was a contemporary and friend of the Delft artist Johannes Vermeer (1632–1675) who pioneered the use of light and shade in art at the same time that van Leeuwenhoek was exploring the use of light to discover the microscopic world. Despite van Leeuwenhoek's efforts, which included the discovery of microorganisms and protozoa, red blood cells and spermatozoa, it was to be another 150 years before, in 1838, the botanist Matthias Schleiden and the zoologist Theodor Schwann formally proposed that all living organisms are composed of cells. Their “cell theory”, which nowadays seems so obvious, was a milestone in the development of modern biology. Nevertheless general acceptance took many years, in large part because the plasma membrane (Fig. 1.2), the membrane surrounding the cell that divides the living inside from the nonliving extracellular medium, is too thin to be seen using a light microscope.
Principles of Microscopy
Microscopes make small objects appear bigger. A light microscope will magnify an image up to 1500 times its original size. Electron microscopes can achieve magnifications up to several million times. However, bigger is only better when more details are revealed. The fineness of detail that a microscope can reveal is its resolving power. This is defined as the smallest distance that two objects can approach one another yet still be recognized as being separate. The resolution that a microscope achieves is mainly a function of the wavelength of the illumination source it employs. The smaller the wavelength, the smaller the object that will cause diffraction, and the better the resolving power. The light microscope, because it uses visible light of wavelength around 500 nanometers (nm; 1 nm = 1/1000 μm), can distinguish objects as small as about half this: 250 nm. It can therefore be used to visualize the smallest cells and the major intracellular structures or organelles. The microscopic study of cell structure and organization is known as cytology. An electron microscope is required to reveal the ultrastructure (the fine detail) of the organelles and other intracellular structures (Fig. 1.2). The wavelength of an electron beam is about 100,000 times less than that of white light. In theory, this should lead to a corresponding increase in resolution. In practice, the transmission type of electron microscope can distinguish structures about 1000 times smaller than is possible in the light microscope, that is, down to about 0.2 nm in size.
The Light Microscope
A light microscope (Figs. 1.3A and 1.4) consists of a light source, which may be the sun or an artificial light, plus three glass lenses: a condenser lens to focus light on the specimen, an objective lens to form the magnified image, and a projector lens, usually called the eyepiece, to convey the magnified image to the eye. Depending on the focal length of the various lenses and their arrangement, a given magnification is achieved. In bright-field microscopy, the image that reaches the eye consists of the colors of white light minus those absorbed by the cell. Most living cells have little color and are therefore largely transparent to transmitted light. This problem can be overcome by cytochemistry, the use of colored stains to selectively highlight particular structures and organelles. However, many of these compounds are highly toxic and to be effective they often require that the cell or tissue is subjected to a series of harsh chemical treatments. A different approach, and one that can be applied to living cells, is the use of phase-contrast microscopy. This relies on the fact that light travels at different speeds through regions of the cell that differ in composition. The phase-contrast microscope converts these differences in refractive index into differences in contrast, and considerably more detail is revealed (Fig. 1.5). Light microscopes come in a number of physical orientations (upright, inverted, etc.) but whatever the orientation of the microscope, the optical principles are the same.
Example 1.1 Sterilization by Filtration
Because even the smallest cells are larger than 1 μm, harmful bacteria and other organisms can be removed from drinking water by passing through a filter with 200 nm diameter holes. Filters can vary in size from huge, such as those used in various commercial processes, to small enough to be easily transportable by backpackers. Filtering drinking water greatly reduces the chances of bringing back an unwanted souvenir from your camping trip!
The Electron Microscope
The most commonly used type of electron microscope in biology is called the transmission electron microscope because electrons are transmitted through the specimen to the observer. The transmission electron microscope has essentially the same design as a light microscope, but the lenses, rather than being glass, are electromagnets that bend beams of electrons (Fig. 1.3B). An electron gun generates a beam of electrons by heating a thin, V-shaped piece of tungsten wire to 3000°C. A large voltage accelerates the beam down the microscope column, which is under vacuum because the electrons are slowed and scattered if they collide with air molecules. The magnified image can be viewed on a fluorescent screen that emits light when struck by electrons. While the electron microscope offers great improvements in resolution, electron beams are potentially highly destructive, and biological material must be subjected to a complex processing schedule before it can be examined. The preparation of cells for electron microscopy is summarized in Figure 1.6. The transmission electron microscope produces a detailed image but one that is static, two-dimensional, and highly processed (Fig. 1.7). Often, only a small region of what was once a dynamic, living, three-dimensional cell is revealed. Moreover, the picture revealed is essentially a snapshot taken at the particular instant that the cell was killed. Clearly, such images must be interpreted with great care. Also, electron microscopes are large, expensive and require a skilled operator. Nevertheless, they are the main source of information on the ultrastructure of the cell at the nanometer scale.
The Scanning Electron Microscope
Whereas the image in a transmission electron microscope is formed by electrons transmitted through the specimen, in the scanning electron microscope it is formed from electrons that are reflected back from the surface of a specimen as the electron beam scans rapidly back and forth over it (Fig. 1.3C). These reflected electrons are detected and used to generate a picture on a display monitor. The scanning electron microscope operates over a wide magnification range, from 10 times to 100,000 times, and has a wide depth of focus. The images created give an excellent impression of the three-dimensional shape of objects (Fig. 1.8). The scanning electron microscope is therefore particularly useful for providing topographical information on the surfaces of cells or tissues. Modern instruments have a resolution of about 1 nm.
IN DEPTH 1.1 FLUORESCENCE MICROSCOPY
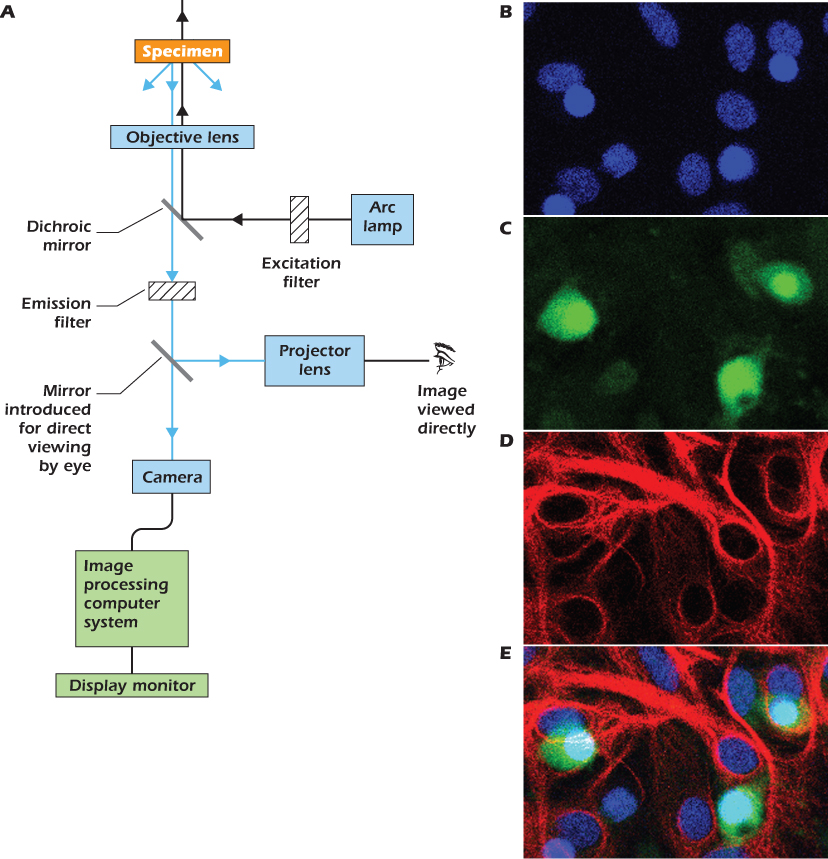
Fluorescent molecules emit light when they are illuminated with light of a shorter wavelength. Familiar examples are the hidden signature in bank passbooks, which is written in fluorescent ink that glows blue (wavelength about 450 nm) when illuminated with ultraviolet light (UV) (wavelength about 360 nm), and the whitener in fabric detergents that causes your white shirt to glow blue when illuminated by the ultraviolet light in a club. The fluorescent dye Hoechst 33342 has a similar wavelength dependence: it is excited by UV light and emits blue light. However, it differs from the dyes used in ink or detergent in that it binds tightly to the DNA in the nucleus and only fluoresces when so bound. Diagram A shows the optical path through a microscope set up so as to look at a preparation stained with Hoechst. White light from an arc lamp passes through an excitation filter that allows only UV light to pass. This light then strikes the heart of the fluorescent microscope: a special mirror called a dichroic mirror that reflects light of wavelengths shorter than a designed cutoff but transmits light of longer wavelength. To view Hoechst, we use a dichroic mirror of cutoff wavelength 400 nm, which therefore reflects the UV excitation light up through the objective lens and onto the specimen. Any Hoechst bound to DNA in the preparation will emit blue light. Some of this will be captured by the objective lens and, because its wavelength is greater than 400 nm, will not be reflected by the dichroic mirror but will instead pass through. An emission filter, set to pass only blue light, cuts out any scattered UV light. The blue light now passes to the eye or camera in the usual way. Image B shows a field of cells cultured from rat brain after staining with Hoechst. Only the nuclei are seen, as bright ovals.
Although some of the structures and chemicals found in cells can be selectively stained by specific fluorescent dyes, others are most conveniently revealed by using antibodies. In this technique an animal (usually a mouse, rabbit, or goat) is injected with a protein or other chemical of interest. The animal's immune system recognizes the chemical as foreign and generates antibodies that bind to (and therefore help neutralize) the chemical. Some blood is then taken from the animal and the antibodies purified. The antibodies can then be labeled by attaching a fluorescent dye. Images C and D show the same field of brain cells but with the excitation filter, dichroic mirror, and emission filter changed so as to reveal in C a protein called ELAV that is found only in nerve cells; and in D, an intermediate filament protein found only in glial cells. The antibody that binds to ELAV is labeled with a fluorescent dye that is excited by blue light and emits green light. The antibody that binds to the glial filaments is labeled with a dye that is excited by green light and emits red light. Because these wavelength characteristics are different, the location of the three chemicals—DNA, ELAV, and intermediate filament—can be revealed independently in the same specimen. Image E shows the previous three images superimposed.
The technique just described is primary immunofluorescence and requires that the antibody to the chemical of interest be labeled with a dye. Only antibodies to chemicals that many laboratories study are so labeled. In order to reveal other chemicals, scientists use secondary immunofluorescence. In this approach, a commercial company injects an animal (e.g., a goat) with an antibody from another animal (e.g., a rabbit). The goat then makes “goat anti-rabbit” antibody. This, the secondary antibody, is purified and labeled with a dye. All the scientist has to do is make or buy a rabbit antibody that binds to the chemical of interest. No further modification of this specialized primary antibody is necessary. Once the primary antibody has bound to the specimen and excess antibody rinsed off, the specimen is then exposed to the fluorescent secondary antibody that binds selectively to the primary antibody. Viewing the stained preparation in a fluorescence microscope then reveals the location of the chemical of interest. The same dye-labeled secondary antibody can be used in other laboratories or at other times to reveal the location of many different chemicals because the specificity is determined by the unlabeled primary antibody.
A completely different approach uses genetically encoded fluorescent molecules. The first to be used was green fluorescent protein from the jellyfish Aequorea victoria. Cells can be induced to make this protein and the living cell viewed by fluorescence microscopy. If the cell is induced to make a chimaeric protein comprising green fluorescent protein fused to some or all of a protein of interest, then fluorescence microscopy can be used to follow the changing location of this protein within a living cell (e.g., Fig. 18.13 ). Green fluorescent protein is described in more detail later (pages 115, 148).
IN DEPTH 1.2 MICROSCOPY REWARDED
Such has been the importance of microscopy to developments in biology that two scientists have been awarded the Nobel prize for their contributions to microscopy. Frits Zernike was awarded the Nobel prize for physics in 1953 for the development of phase-contrast microscopy whilst Ernst Ruska received the same award in 1986 for the invention of the transmission electron microscope. Ruska's prize marks one of the longest gaps between a discovery (in the 1930s, in the research labs of the Siemens Corporation in Berlin) and the award of a Nobel prize. Anton van Leeuwenhoek died almost two centuries before the Nobel prizes were introduced in 1901 and the prize is not awarded posthumously.
Only Two Types of Cell
Superficially at least, cells exhibit a staggering diversity. Some lead a solitary existence; others live in communities; some have defined, geometric shapes; others have flexible boundaries; some swim, some crawl, and some are sedentary. Given these differences, it is perhaps surprising that there are only two types of cell (Fig. 1.9). Prokaryotic (Greek for “before nucleus”) cells have very little visible internal organization so that, for instance, the genetic material, stored in the molecule deoxyribonucleic acid (DNA), is free within the cell. They are especially small, the vast majority being 1–2 μm in length. The prokaryotes are made up of two broad groups of organisms, the bacteria and the archaea (Fig. 1.10). The archaea were originally thought to be an unusual group of bacteria but we now know that they are a distinct group of prokaryotes with an independent evolutionary history. The cells of all other organisms, from yeasts to plants to worms to humans, are eukaryotic (Greek for “with a nucleus”). These are generally larger (5–100 μm, although some eukaryotic cells are large enough to be seen with the naked eye; see Fig. 1.1) and structurally more complex. Eukaryotic cells contain a variety of specialized structures known collectively as organelles, surrounded by a viscous substance called cytosol. Their DNA is held within the largest organelle, the nucleus. The structure and function of organelles will be described in detail in subsequent chapters. Table 1.1 summarizes the differences between prokaryotic and eukaryotic cells.
Table 1.1 Differences between Prokaryotic and Eukaryotic Cells
Size |
Usually 1–2 μm |
Usually 5–100 μm |
Nucleus |
Absent |
Present, bounded by nuclear envelope |
DNA |
Usually a single circular molecule (=chromosome) |
Multiple linear molecules (chromosomes)a |
Cell division |
Simple fission |
Mitosis or meiosis |
Internal membranes |
Rare |
Complex (nuclear envelope, Golgi apparatus, endoplasmic reticulum, etc.) |
Ribosomes |
70Sb |
80S (70S in mitochondria and chloroplasts) |
Cytoskeleton |
Rudimentary |
Microtubules, microfilaments, intermediate filaments |
Motility |
Rotary motor (drives bacterial flagellum) |
Dynein (drives cilia and flagella); kinesin, myosin |
First appeared |
3.5 × 109 years ago |
1.5 × 109 years ago |
Cell Division
One of the major distinctions between prokaryotic and eukaryotic cells is their mode of division. In prokaryotes the circular chromosome is duplicated from a single replication origin by a group of enzymes that reside on the inside of the plasma membrane. At the completion of replication the old and new copies of the chromosome lie side by side on the plasma membrane, which then pinches inwards between them. This process, which generates two equal, or roughly equal, progeny cells is described as binary fission. In eukaryotes the large, linear chromosomes, housed in the nucleus, are duplicated from multiple origins of replication by enzymes located in the nucleus. Some time later the nuclear envelope breaks down and the replicated chromosomes are compacted so that they can be segregated without damage during mitosis. We will deal with mitosis in detail in Chapter 18. For the moment we should be aware that although it is primarily about changes to the nucleus, mitosis is accompanied by dramatic changes to the organization of the rest of the cell. A new structure, the mitotic spindle, is assembled specifically to move the chromosomes apart while other structures such as the Golgi apparatus and endoplasmic reticulum are dismantled so that their components can be divided among the two progeny cells following cell division.
Viruses
Viruses occupy a unique position between the living and nonliving worlds. On the one hand they are made of the same molecules as living cells. On the other they are incapable of independent existence, being completely dependent on a host cell for reproduction. Almost all living organisms have viruses that infect them. Human viruses include polio, influenza, herpes, rabies, ebola, smallpox, chickenpox, and HIV (human immunodeficiency virus, the causative agent of AIDS). Viruses are submicroscopic particles consisting of a core of genetic material enclosed within a protein coat called the capsid. Some have an extra membrane layer called the envelope. Viruses are metabolically inert until they enter a host cell, whereupon their genetic material directs the host cell machinery to produce viral protein and viral genetic material. Viruses often insert their genome into that of the host, an ability that is widely made use of in molecular biology research (Chapter 7). Bacterial viruses, bacteriophages, are used by scientists to transfer genes between bacterial strains. Human viruses are used as vehicles for gene therapy. By exploiting the natural infection cycle of a virus such as adenovirus, it is possible to introduce a functional copy of a human gene into the cells of a patient suffering from a genetic disease such as Leber congenital amaurosis .
Origin of Eukaryotic Cells
Prokaryotic cells are simpler in their organization than eukaryotic cells and are assumed to be more primitive. According to the fossil record, prokaryotic organisms antedate, by at least 2 billion years, the first eukaryotes that appeared some 1.5 billion years ago. It seems highly likely that eukaryotes evolved from prokaryotes, and the most likely explanation of this process is the endosymbiotic theory. The basis of this theory is that some eukaryotic organelles originated as free-living bacteria that were engulfed by larger cells in which they established a mutually beneficial relationship. For example, mitochondria would have originated as free-living aerobic bacteria and chloroplasts as cyanobacteria, which are photosynthetic prokaryotes formerly known as blue-green algae. The endosymbiotic theory provides an attractive explanation for the fact that mitochondria and chloroplasts contain their own DNA and ribosomes, both of which are more closely related to those of bacteria than to all the other DNA and ribosomes in the same cell. The case for the origin of other eukaryotic organelles is less persuasive. Nevertheless, while it is clearly not perfect, most biologists are now prepared to accept that the endosymbiotic theory provides at least a partial explanation for the evolution of the eukaryotic cell from prokaryotic ancestors.
Cell Specialization in Animals
Animals are multicellular communities of individual cells. Lying between and supporting the cells is the extracellular matrix (Fig. 1.7) of different types of fiber around which the fluids and solute of the interstitial fluid can easily pass. All the body cells that comprise a single organism share the same set of genetic instructions in their nuclei (with the single exception of lymphocytes, ). Nevertheless, the cells are not all identical. Rather, they form a variety of tissues, groups of cells that are specialized to carry out a common function. This specialization occurs because different cell types read out different parts of the DNA blueprint and therefore make different proteins. In animals there are four major tissue types: epithelium, connective tissue, nervous tissue, and muscle. Some examples of the cells that make up these tissues are shown in Figure 1.11.
Epithelia are sheets of cells that cover the surface of the body and line its internal cavities such as the lungs and intestine. The cells may be columnar, taller than they are broad (Fig. 1.11B), or squamous, meaning flat (e.g., the capillary cell in Fig. 1.7). They are often polarized, meaning that one surface of the cell is distinct in its organization, composition and appearance from the other. In the intestine, the single layer of columnar cells lining the inside, or lumen, has an absorptive function that is increased by the folding of the surface into villi (Fig. 1.12). The luminal surfaces of these polarized cells have microvilli that increase the surface area even further. The basal (bottom) surface sits on a thin planar sheet of specialized extracellular matrix called the basement membrane or basal lamina. Many of the epithelial cells of the airways, for instance, those lining the trachea and bronchioles, have cilia on their surfaces (Fig. 1.8). These are hairlike appendages that actively beat back and forth, moving a layer of mucus away from the lungs (Chapter 17). Particles and bacteria are trapped in the mucus layer, preventing them from reaching the delicate air exchange membranes in the lung. In the case of the skin, the epithelium is said to be stratified because it is composed of several layers.
Connective tissues provide essential support for the other tissues of the body. They include bone, cartilage, and adipose (fat) tissue. Unlike other tissues, connective tissue contains relatively few cells within a large volume of extracellular matrix that consists of different types of fiber embedded in amorphous ground substance (Fig. 1.12). The most abundant of the fibers is collagen, a protein with the tensile properties of steel that accounts for about a third of the protein of the human body. Other fibers have elastic properties that permit the supported tissues to be displaced and then to return to their original position. The amorphous ground substance absorbs large quantities of water, facilitating the diffusion of metabolites, oxygen, and carbon dioxide to and from the cells in other tissues and organs. Of the many cell types found in connective tissue, two of the most important are fibroblasts, which make and secrete the ground substance and fibers, and macrophages, which remove foreign, dead, and defective material. A number of inherited diseases are associated with defects in connective tissue. Marfan's syndrome, for example, is characterized by long arms, legs, and torso and by a weakness of the cardiovascular system and eyes. These characteristics result from a defect in the organization of the collagen fibers.
Nervous tissue is a highly modified epithelium that is composed of several cell types. Principal among these are the nerve cells, also called neurons (Fig. 1.11C), along with a variety of supporting cells that help maintain them. Neurons extend processes called axons, which can be over a meter in length. Neurons constantly monitor what is occurring inside and outside the body. They integrate and summarize this information and mount appropriate responses to it (Chapters 14–16). Another type of cell, glia, has other roles in nervous tissue including forming the electrical insulation around axons.
Muscle tissue can be of two types, smooth or striated. Smooth muscle cells are long and slender and are usually found in the walls of tubular organs such as the intestine and many blood vessels. In general, smooth muscle cells contract slowly and can maintain the contracted state for a long period of time. There are two classes of striated muscle: cardiac and skeletal. Cardiac muscle cells (Fig. 1.7) make up the walls of the heart chambers. These are branched cells that are connected electrically by gap junctions , and their automatic rhythmical contraction powers the beating of the heart. Each skeletal muscle is a bundle of hundreds to thousands of fibers, each fiber being a giant single cell with many nuclei. This rather unusual situation is the result of an event that occurs in the embryo when the cells that give rise to the fibers fuse together, pooling their nuclei in a common cytoplasm (the term cytoplasm is historically a crude term meaning the semi-viscous ground substance of cells; we use the term to mean everything inside the plasma membrane except the nucleus). The mechanism of muscle contraction will be described in Chapter 17.
Stem Cells and Tissue Replacement
Cells multiply by division. In the human body an estimated 25 million cell divisions occur every second! These provide new cells for the blood and immune systems, for the repair of wounds and the replacement of dead cells. In complex tissues such as those described above, division is restricted to a small number of stem cells from which all of the other cells of the tissue derive. In the case of the intestine, folds in the surface epithelium form crypts, each of which contains approximately 250 cells (Fig. 1.12). Mature cells at the top die and must be replaced by the division of between four and six stem cells near the base of the crypt. Each stem cell divides roughly twice a day, the resulting cells moving up the crypt to replace those lost at the surface. Benign (noncancerous) polyps can be formed in the intestine if this normal balance between birth and death is disturbed.
As in the intestine, stem cells in other tissues exist in specific locales, called niches, with environments that support their special and vital functions. In many tissues the requirement to replace dead cells is much less than it is in the intestine and in such cases the stem cell niche must maintain its occupants in a quiescent (nondividing) state until needed. Like stem cells themselves, the properties of niches remain deeply mysterious. For the moment we have few markers that allow us to specifically distinguish stem cells from the cells around them and hence unambiguously identify the territories they occupy. The resolution of such questions is vital if the potential of stem cells in cell therapy is to be realized.
IN DEPTH 1.3 Stem Cells
Few developments in modern biology have had the medical and social impact that has followed the discovery of stem cells. These are unspecialized cells from which all specialized cells in the human body are derived. They are present in small numbers in almost all tissues and they can either remain unspecialized for long periods, dividing at intervals to produce more stem cells (a process called self-renewal) or they can differentiate into a wide variety of specialized cell types. This means that stem cells have enormous potential in what has become known as stem cell therapy. This involves treating patients suffering from spinal cord injury or from a stroke, for example, with stem cells that have been preprogrammed to replace the damaged tissue.
There are two kinds of stem cells: adult stem cells and embryonic stem cells. It is the latter that has caused much of the controversy about stem cell therapy since they are derived from human embryos that are left over after in vitro fertilization treatment. Cells from a four- to five-day-old human embryo are pluripotent, that is, they can be induced to create just about any cell type. There are fewer ethical objections to the use of adult stem cells, but as yet, these have nothing like the versatility of their embryonic counterparts. Eventually it should be possible to isolate stem cells from a patient and program these appropriately before reintroducing them into the damaged tissue. Because they are the patient's own cells, questions of rejection and other complications do not arise.
In some respects, stem cell therapy is not really new. Bone marrow transplantation has been familiar in the treatment of a variety of blood diseases for more than 40 years. Patients suffering from cancers such as leukemia and lymphoma, for example, receive doses of chemotherapy that kill the stem cells that are located in the bone marrow. These give rise to the different cells in the blood; the red cells that carry oxygen around the body, the white cells that help fight infection, and the platelets that help blood clot. Replacement bone marrow, usually from a healthy relative, is therefore essential to restock these stem cells and allow the patient to make a full recovery.
The Cell Wall
Many types of cell, particularly bacteria and plant cells, create a rigid case around themselves called a cell wall. For cells that live in an extracellular medium more dilute than their own cytosol, the cell wall is critical in preventing the cell bursting. For example, penicillin and many other antibiotics block the synthesis of bacterial cell walls with the result that the bacteria burst. Within trees, plant cells modify the cell wall to generate the woody trunk. Animal cells do not have cell walls.
Summary
1. All living organisms are made of cells.
2. Our understanding of cell structure and function has gone hand in hand with developments in microscopy and its associated techniques.
3. Light microscopy revealed the diversity of cell types and the existence of the major organelles such as the nucleus and mitochondria.
4. The electron microscope reveals the detailed structure of the larger organelles and resolves the cell ultrastructure, the fine detail, at the nanometer scale.
5. There are only two types of cells, prokaryotic and eukaryotic.
6. Prokaryotic cells have little visible internal organization. They are usually 1–2 μm in size.
7. Eukaryotic cells usually measure 5–100 μm. They contain a variety of specialized internal organelles, the largest of which, the nucleus, contains the genetic material.
8. The endosymbiotic theory proposes that some eukaryotic organelles, such as mitochondria and chloroplasts, originated as free-living prokaryotes.
9. In multicellular organisms, cells are organized into tissues. In animals there are four tissue types: epithelium, connective tissue, nervous tissue, and muscle.
10. The extracellular matrix is found on the outside of animal cells.
11. In tissues, specialized cells arise from unspecialized stem cells.
REVIEW QUESTIONS
We use the same format of review questions throughout the book. For each of the numbered questions choose the best response from the lettered list. The same response may apply to more than one numbered question. Unless specifically told to do so, you should not refer back to the chapter text or figures in answering the questions. Answers are at the back of the book, starting on .
1.1 Theme: Dimensions in Cell Biology
A. 0.025 nm
B. 0.2 nm
C. 20 nm
D. 250 nm
E. 2,000 nm
F. 20,000 nm
G. 200,000 nm
H. 5,000,000 nm
I. 1,000,000,000 nm
J. 20,000,000,000 nm
From the above list, select the dimension most appropriate for each of the descriptions below.
1. A typical bacterium
2. A typical eukaryotic cell
3. The longest cell in the human body
4. Resolution of a light microscope
5. Resolution of a transmission electron microscope
1.2 Theme: Types of Cell
A. bacterium
B. epithelial cell
C. fibroblast
D. macrophage
E. glial cell
F. skeletal muscle cell
G. stem cell
From the above list of cell types, select the cell corresponding to each of the descriptions below.
1. A cell that synthesizes collagen
2. A cell type found in nervous tissue
3. A cell type that forms sheets, e.g., to separate different spaces in the body
4. A cell whose role is to remove dead and foreign material
5. A cell with no nuclear envelope
6. Large cells with multiple nuclei
7. Undifferentiated cells capable of multiple rounds of cell division
1.3 Theme: Some Basic Components of the Eukaryotic Cell
3. Identify each of the cellular components below from the figure above.
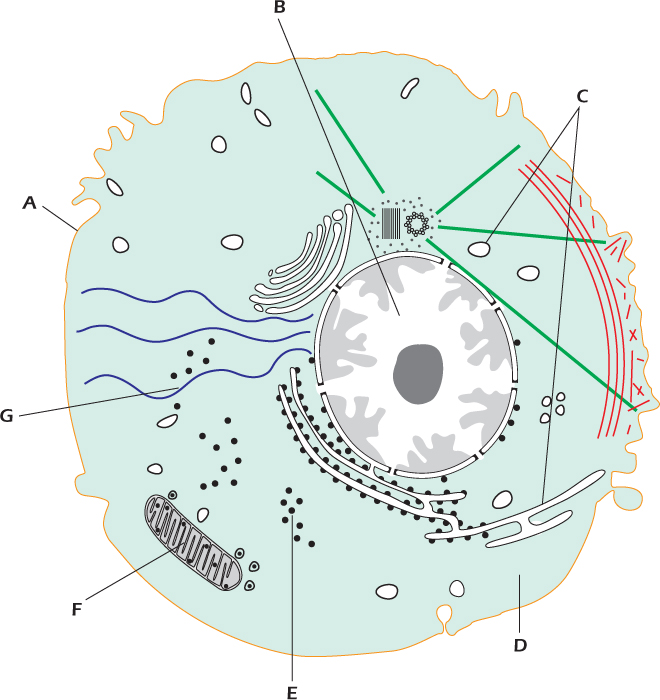
1. Cytosol
2. Internal membranes
3. Mitochondrion
4. Nucleus
5. Plasma membrane
THOUGHT QUESTION
Each chapter has a thought question. For these you are encouraged to refer back to the text and diagrams within the chapter to formulate your response. Answers appear earlier in the relevant chapter, printed upside down.
You wish to test the hypothesis that a particular inherited human disease is characterized by malformation of the Golgi apparatus. What type of microscopy would you use to examine a tissue biopsy from a patient?
Further Reading
Booth, C., and Potten, C. S. (2000) Gut instincts: thoughts on intestinal epithelial stem cells. Journal of Clinical Investigation 105, 1493–1499.
Gest, H. (2004) The discovery of microorganisms by Robert Hooke and Antoni van Leeuwenhoek, Fellows of the Royal Society. Notes and Records of the Royal Society of London, 58, 187–201.
Harris, H. (1999) The Birth of the Cell, Yale University Press, New Haven, Connecticut.