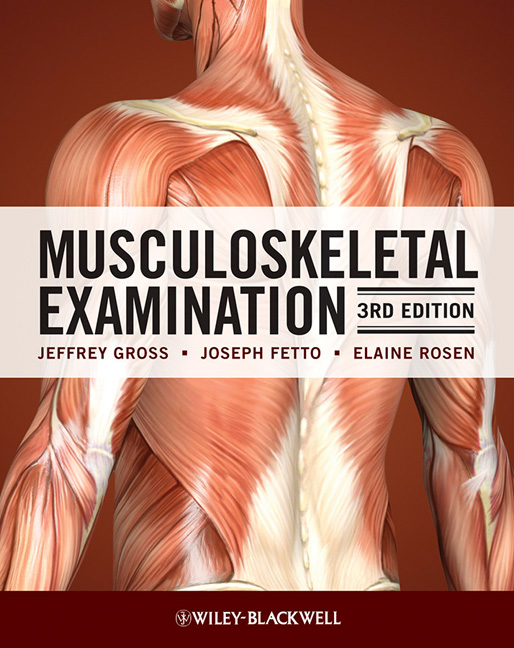
Contents
This edition first published 2009 © 1996, 2002, 2009 by Jeffrey Gross, Joseph Fetto, Elaine Rosen
Blackwell Publishing was acquired by John Wiley & Sons in February 2007. Blackwell’s publishing program has been merged with Wiley’s global Scientific, Technical and Medical business to form Wiley-Blackwell.
Registered office: John Wiley & Sons Ltd, The Atrium, Southern Gate, Chichester, West Sussex, PO19 8SQ, UK
Editorial offices: 9600 Garsington Road, Oxford, OX4 2DQ, UK
The Atrium, Southern Gate, Chichester, West Sussex, PO19 8SQ, UK
111 River Street, Hoboken, NJ 07030-5774, USA
For details of our global editorial offices, for customer services and for information about how to apply for permission to reuse the copyright material in this book please see our website at
The right of the author to be identified as the author of this work has been asserted in accordance with the Copyright, Designs and Patents Act 1988.
All rights reserved. No part of this publication may be reproduced, stored in a retrieval system, or transmitted, in any form or by any means, electronic, mechanical, photocopying, recording or otherwise, except as permitted by the UK Copyright, Designs and Patents Act 1988, without the prior permission of the publisher.
Wiley also publishes its books in a variety of electronic formats. Some content that appears in print may not be available in electronic books.
Designations used by companies to distinguish their products are often claimed as trademarks. All brand names and product names used in this book are trade names, service marks, trademarks or registered trademarks of their respective owners. The publisher is not associated with any product or vendor mentioned in this book. This publication is designed to provide accurate and authoritative information in regard to the subject matter covered. It is sold on the understanding that the publisher is not engaged in rendering professional services. If professional advice or other expert assistance is required, the services of a competent professional should be sought.
Library of Congress Cataloging-in-Publication Data
Gross, Jeffrey M., 1957–
Musculoskeletal examination / Jeffrey M. Gross, Joseph Fetto, Elaine Rosen.—3rd ed.
p. ; cm.
Includes bibliographical references and index.
ISBN 978-1-4051-8049-8
1. Musculoskeletal system—Examination. I. Fetto, Joseph. II. Rosen, Elaine. III. Title. [DNLM: 1. Musculoskeletal Diseases—diagnosis. 2. Musculoskeletal Physiology. 3. Musculoskeletal System—anatomy & histology. 4. Physical Examination—methods.
WE 141 G878m 2009]
RC925.7.G76 2009
616.70076–dc22
2008039510
ISBN: 978-1-4051-8049-8
A catalogue record for this book is available from the British Library.
How to Use This Book
Musculoskeletal Examination is to be used as both a teaching text and a general reference on the techniques of physical examination. This volume represents the joint authoring efforts of a physiatrist, an orthopedic surgeon, and a physical therapist and presents the information in a clear and concise format, free of any professional biases that reflect one specialty’s preferences. The importance of this will be seen as we take you through each anatomical region and delineate the basic examination. Included in each chapter are the abnormalities most frequently encountered noted while performing an examination.
The book is organized into regional anatomical sections including the spine and pelvis, the upper extremity, and the lower extremity. The book opens with two chapters that define the structures of the musculoskeletal system and discuss the basic concepts and parts of the musculoskeletal exam. A final chapter describes the examination of gait.
Each main chapter is organized in an identical manner:
In Chapter 2, Basic Concepts of the Physical Examination, we provide you with a framework for performing the examination, beginning with observation and ending with palpation. However, in each regional anatomy chapter, palpation follows observation and subjective examination and precedes all other sections. This is deliberate. For reasons of length, we felt it important to discuss each anatomical region and its own special anatomical structures as soon as possible in each chapter. This avoids repetition, gives you the anatomy early in each chapter, and then allows you to visualize each structure as you read the subsequent sections on testing. Hopefully, this will reinforce the anatomy and help you apply anatomy to function and function to the findings of your examination.
Each chapter includes a generous number of original line drawings, many of which are two color. These provide clear snapshots of how to perform each examination technique. Thirty-two x-rays and MRIs have been included to help you with radiological anatomy. Paradigms and tables provide additional information that will help you understand the how and why of each examination technique.
By using Musculoskeletal Examination as a guide and reference, the reader will be able to perform the complete basic examination and understand common abnormalities and their pathological significance. We hope that our readers will gain an appreciation for the intimate relationship between the structure and function of the components of the musculoskeletal system. This understanding should then enable any reader to make a correct diagnosis and a successful treatment plan for each patient.
Acknowledgments
The writing of Musculoskeletal Examination would not have been possible without the overwhelming support and understanding of my wife Elizabeth and my sons, Tyler and Preston. I also want to thank my parents, Malcolm and Zelda Gross, as well as my teachers Dr. Joseph Goodgold, Dr. Bruce Grynbaum, Dr. Howard Thistle, and Dr. Matthew Lee for their guidance and efforts on my behalf.
J.G.
Thank you to my wife and family for their understanding, patience, support, and love.
J.F.
To my husband, Jed, for his unlimited patience, understanding, and encouragement.
To my business partner and friend, Sandy, for being there whenever I needed her.
To my family for their support, and to my many patients, colleagues, and friends who have helped me grow.
E.R.
CHAPTER 1: Introduction
The intention of this book is to provide the reader with a thorough knowledge of regional anatomy and the techniques of physical examination. A second and equally important intention is to describe a method for the interpretation and logical application of the knowledge obtained from a physical examination.
What Is a Physical Examination?
The physical examination is the inspection, palpation, measurement, and auscultation of the body and its parts. It is the step that follows the taking of a patient history and precedes the ordering of laboratory tests and radiological evaluation in the process of reaching a diagnosis.
What Is the Purpose of the Physical Examination?
The physical examination has two distinct purposes. The first is to localize a complaint, that is, to associate a complaint with a specific region and, if possible, a specific anatomical structure. The second purpose of a physical examination is to qualify a patient’s complaints. Qualifying a complaint involves describing its character (i.e., dull, sharp, etc.), quantifying its severity (i.e., visual analog scale; grade I, II, III), and defining its relationship to movement and function.
How Is the Physical Examination Useful?
By relating a patient’s complaints to an anatomical structure, the physical examination brings meaning to a patient’s history and symptoms. This, however, presupposes that the clinician possesses a thorough knowledge of anatomy. It also requires a methodology for the logical analysis and application of the information obtained from the patient’s history and physical examination. This methodology is derived from a clinical philosophy based on specific concepts. These concepts are as follows:
1. If one knows the structure of a system and understands its intended function, it is possible to predict how that system is vulnerable to breakdown and failure (injury).
2. A biological system is no different from an inorganic system in that it is subject to the same laws of nature (physics, mechanics, engineering, etc.). However, the biological system, unlike the inorganic system, has the potential not only to respond but also to adapt to changes in its environment.
Such concepts lay the foundation for understanding the information obtained on physical examination. They also lead to a rationale for the treatment and rehabilitation of injuries. A correlation of this type of analysis is that it becomes possible to anticipate injuries. This in turn permits proactive planning for the prevention of injuries.
How Does the Musculoskeletal System Work?
The musculoskeletal system, like any biological system, is not static. It is in a constant state of dynamic equilibrium. This equilibrium is termed homeostasis.
As such, when subjected to an external force or stress, a biological system will respond in a very specific manner. Unlike the inorganic system (i.e., an airplane wing that is doomed to fail after a predictable number of cycles of load), the biological system will attempt to reestablish an equilibrium state in response to a change that has occurred in its environment. In doing so, the biological system will experience one of three possible scenarios: adaptation (successful establishment of a new equilibrium state without breakdown), temporary breakdown (injury), or ultimate breakdown (death). These scenarios can be expressed graphically. Any system can be stressed in one of two modes: acute single supratolerance load or chronic repetitive submaximal tolerance load (). In the first mode, the system that suffers acute failure is unable to resist the load applied. In the second mode, the system will function until some fatigue limit is reached, at which time failure will occur. In the biological system, either failure mode will initiate a protective-healing response, termed the inflammatory reaction. The inflammatory reaction is composed of cellular and humoral components, each of which initiates a complex series of neurological and cellular responses to the injury. An important consequence of the inflammatory reaction is the production of pain. The sole purpose of pain is to bring one’s attention to the site of injury. Pain prevents further injury from occurring by causing protective guarding and limited use of the injured structure. The inflammatory response is also characterized by increased vascularity and swelling in the area of injury. These are the causes of the commonly observed physical signs (i.e., redness and warmth) associated with the site of injury.
Biological systems, like inorganic systems, can fail under one of two modes: an acute single supramaximal stress or repetitive submaximal chronic loading.
However, the problem with pain is that although it brings protection to the area of injury (the conscious or unconscious removal of stress from the injured area), and permits healing to take place by removing dynamic stimuli from the biological system, this removal of stimuli (rest) promotes deterioration of a system’s tolerance limit to a lower threshold. In this way, when the injury has resolved, the entire system, although “healed,” may actually be more vulnerable to reinjury when “normal” stresses are applied to the recently repaired structures. This initiates the “vicious cycle of injury” ().
Contrary to this scenario is one in which the biological system successfully adapts to its new environment before failure occurs. This situation represents conditioning of a biological system. The result is hypertrophy, enhanced function, and a consequent increase in the system’s tolerance limit. The concept acting here is that the biological system’s tolerance limit will adapt to increased demands if the demands are applied at a frequency, intensity, and duration within the system’s ability to adapt ().
The “vicious cycle of injury” results from the reinjury of a vulnerable, recently traumatized system. This increased vulnerability occurs due to a diminishing of a system’s tolerance limit as a result of adaptation to a lower level of demand during the period of rest necessitated by pain.
Therefore, during the physical examination, asymmetry must be noted and analyzed as representing either adaptation or deconditioning of a given system. Any of these fundamental principles under which the musculoskeletal system functions makes it possible to organize the information obtained from a physical examination and history into general categories or pathological conditions (traumatic, inflammatory, metabolic, etc.), and the subsets of these conditions (tendinitis, ligamentous injuries, arthritis, infection, etc.). From such an approach, generalizations called paradigms can be formulated. These paradigms provide a holistic view of a patient’s signs and symptoms. In this way, diagnoses are arrived at based on an analysis of the entire constellation of signs and symptoms with which a given patient presents. This method, relying on a multitude of factors and their interrelationships rather than on a single piece of information, such as the symptom of clicking or swelling, ensures a greater degree of accuracy in formulating a diagnosis.
What Are Paradigms?
Paradigms are snapshots of classic presentations of various disease categories. They are, as nineteenthcentury clinicians would say, “augenblick,” a blinkof- the-eye impression of a patient (). From such an impression, a comparison is made with an idealized patient, to evaluate for congruities or dissimilarities. Here is an example of a paradigm for osteoarthritis: a male patient who is a laborer, who is at least 50 years old, whose complaints are asymmetrical pain involving larger joints, and whose symptoms are in proportion to his activity. Another example might be that of rheumatoid arthritis. This paradigm would describe a female patient who is 20–40 years old, complaining of symmetrical morning stiffness involving the smaller joints of the hands, with swelling, possibly fever, and stiffness reducing with activity.
Paradigms may also be created for specific tissues (i.e., joints, tendons, muscles, etc.). The paradigm for a joint condition such as osteoarthritis would be welllocalized pain, swelling, stiffness on sedentary posturing, and pain increasing in proportion to use, whereas a paradigm for a mild tendon inflammation (tendinitis) may be painful stiffness after sedentary posturing that becomes alleviated with activity and gentle use. A paradigm for ligament injury would include a history of a specific traumatic event, together with the resultant loss of joint stability demonstrated on active and passive tensile loading of a joint.
The reader is encouraged to create his or her own paradigms for various conditions—paradigms that include the entire portrait of an injury or disease process with which a given patient or tissue may be compared. In this process, it will become obvious that it is not sufficient to limit one’s expertise to the localization of complaints to an anatomical region. It is also necessary to be able to discriminate between the involvement of specific structures that may lie in close proximity within that region (i.e., bursae and tendons overlying a joint).
It can be concluded therefore that an accurate physical examination is as critical to the process of diagnosis as is a complete and accurate history of a patient’s complaints. An accurate physical examination demands a thorough knowledge and familiarity with anatomy and function.
Conditioning is the adaptation of a biological system to the controlled application of increasing stress at a frequency, intensity, and duration within the system’s tolerance limit, with a resultant increase in the system’s tolerance limit.
What Are the Components of the Musculoskeletal System?
The musculoskeletal system is composed of bone, cartilage, ligaments, muscle, tendons, synovium, bursae, and fascia. This system is derived embryologically from the mesenchyme and is composed of soft and hard connective tissues. These tissues have evolved to serve two basic functions: structural integrity and stable mobility. The tissues are composite materials made up of cells lying within the extracellular matrix they produce.
Paradigms for osteoarthritis and rheumatoid arthritis.
Paradigm for osteoarthritis | Paradigm for rheumatoid arthritis |
Male | Female |
Laborer | 20–40 years old |
50+ years old | Symmetrical small joint involvement |
Large joint involvement | Associated swelling, fever, rash, morning stiffness |
Asymmetrical involvement | Abating with use |
Pain in proportion to activity |
Collagen, a long linear protein (Figure ), is the most abundant of the extracellular materials found in connective tissues. The foundation of collagen is a repetitive sequence of amino acids that form polypeptide chains. Three such chains are then braided together to form a triple helical strand called tropocollagen. These strands join to make microfibrils; long linear structures specifically designed to resist tensile loading. The microfibrils are bonded together through chemical cross-linking to form collagen fibers. The degree of cross-linking determines the physical properties of a specific collagen fiber. The more cross-linking that exists, the stiffer the fiber will be. The degree of collagen cross-linking is in part genetically and in part metabolically determined. This explains why some people are much more flexible than others. Vitamin C is critical for the formation of cross-links. As such, scurvy, a clinical expression of vitamin deficiency, is characterized by “weak tissues.” Hypermobility of joints (i.e., ability to extend the thumbs to the forearms, ability to hyperextend at the knees and elbows, excessive subtalar pronation with flat, splayed feet) is a clinical manifestation of genetically determined collagen cross-linking ().
(a) Collagen is a linear protein made of α-chains that wind into a triple helix. (b) Collagen fibrils are formed by the cross-linking of collagen monomer proteins. (c) The different types of collagen are determined by the number of α1 and α2 collagen monomers that join to form a triple-helix collagen molecule. For example, two α1 chains and one α2 chain that join to form a triple-helix make type I collagen, which is found in bone, tendon, ligament, fascia, skin, arteries, and the uterus. Type II collagen, which is found in articular cartilage, contains three α1 chains. There are at least 12 different collagen types.
Different types of collagen exist for different categories of tissues. These types are defined by the specific composition of the polypeptide chains that form the strands of the collagen molecules. Type I collagen is found in connective tissue such as bone, tendons, and ligaments. Type II is found uniquely in articular hyaline cartilage. Other collagen types exist as well ().
If collagen represents the fiber in the composite structure of connective tissue, ground substance represents the “filler” between the fibers. The main components of ground substance are aggregates of polyglycan macromolecules. An example of such a macromolecule is the proteoglycan hyaluronic acid, found in articular cartilage. Hyaluronic acid is a molecule of more than 1 million daltons. It is composed of a long central core from which are projected many protein side chains containing negatively charged sulfate radicals. It can best be visualized as a bristle brush from which many smaller bristle brushes are projected (). These strongly negative sulfate radicals make the hyaluronic acid molecule highly hydrophilic (water attracting). This ability to attract and hold water allows the connective tissue ground substance to function as an excellent hydrostatic bearing surface that resists compression load.
Immobilization reduces the diffusion and migration of nutrients throughout the connective tissues. This in turn compromises cellular activity and upsets the normal homeostatic balance of collagen and ground substance turnover. The result is an atrophy of collagen fibers and a diminution of ground substance (Cantu and Grodin, 2001), with subsequent deterioration of the connective-tissue macrofunction (i.e., chondromalacia patellae).
Bone
Bone provides the structure of the body. It is the hardest of all connective tissues. One-third of bone is composed of collagen fibers and two-thirds mineral salts, primarily calcium hydroxyapatite. Bone is formed in response to stress. Although genetically determined, the size and shape of a bone are dependent on environmental factors for its full expression. This response of bone to its loading history has been termed Wolff’s law. There are two major types of bone: cortical and cancellous. All bones are covered by highly vascularized and innervated tissue called periosteum, except when they are within the synovial cavity of a joint ().
The proteoglycan aggregate is formed on a backbone of hyaluronic acid and has the appearance of a bristle brush.
Cortical bone is very dense, highly calcified, and uniquely constructed to resist compression loads. It can also resist tensile bending and torsional loads, but much more poorly. This is a direct function of cortical bone’s ultrastructure, which is a composite of flexible collagen fibers and rigid mineral crystals. Cortical bone is usually found within the diaphysis of long bones. It has a hollow central cavity that is termed the medullary canal or marrow cavity.
At the end of long bones and at the sites of tendon and ligament attachments, bones tend to expand and cortical bone gives way to a more porous structure, termed cancellous or trabecular bone. The trabeculae of cancellous bones lie in the direction of transmitted loads. They act as conduits of load from the articular surface to the underlying diaphyseal cortical bone. Overload of the trabeculae will, on a microscopic scale, duplicate overload of an entire bone (i.e., fracture). This overload, because of the innervation that exists within a bone, will give rise to pain (arthritic discomfort due to mechanical overload secondary to joint deformity or erosion of articular cartilage). The resultant healing of these microfractures leads to increased calcium deposition, hence subchondral sclerosis noted around articular joints on x-ray films, and hypertrophy of stressed sites such as the midshaft of the tibia secondary to stress fractures occurring from overuse in distance running.
The structure of a typical long bone.
Cartilage
Cartilage is a connective tissue made of cells (chondroblasts and chondrocytes) that produce an extracellular matrix of proteoglycans and collagen fibers with a high water content. The tensile strength of cartilage is due to the collagen component. Its resistance to compression is due to the ability of proteoglycan to attract and hold water. Cartilage types include articular or hyaline cartilage (); fibrocartilage, which exists at the attachment sites of ligaments, tendons, and bones; fibroelastic cartilage, found in menisci and intravertebral discs; and growth-plate cartilage, located in the physis of immature bones. With age, cartilage tends to decrease in water content and the number of cross-links among collagen molecules increases. The result is that cartilage tissue becomes more brittle, less supple, and less able to resist tensile, torsional, and compression loading. Hence, cartilage becomes more vulnerable to injury with age.
The composition and structure of articular hyaline cartilage. Water moves in and out of the cartilage due to the pressure of the joint surfaces on one another and attraction of the water by the ground substance. Note the orientation of the collagen fibers.
Articular cartilage lines the spaces in synovial joints. It is attached to the underlying bone by a complex interdigitation analogous to that of a jigsaw puzzle. Regeneration of this cartilage is slow and inconsistent in terms of restoration of articular integrity. It can be replaced by a less mechanically efficient fibrocartilage after injuries have occurred. There are no blood vessels within articular cartilage and nutrition is solely dependent on the loading and unloading of the joint, which allows water-soluble nutrients and waste products to enter and leave the cartilaginous matrix through a porous surface layer.
The fibroelastic cartilage of the intervertebral disc allows for very minimal movement between adjacent vertebrae while providing shock absorption. Because of the orientation of the fibers, they are more vulnerable to flexion and rotational forces. Fibroelastic cartilage is also present in the menisci of the knee. Here, it functions not only to absorb shock but also to increase the functional surface area of the joint, thereby providing additional stability. Because of its elastin content, fibroelastic cartilage is resilient and able to return to its prior shape following deformation.
Ligaments
Ligaments are the static stabilizers of joints. They connect bones to bones (). Ligaments and other capsular structures of the joint are made of dense, organized connective tissue. Ligaments contain collagen and a variable amount of elastin. The collagen provides tensile strength to the ligaments and elastin provides suppleness. The fibers of collagen are arranged more or less parallel to the forces that the ligament is intended to resist. Most ligaments and capsular tissues enter the bone as a progression from collagen fibers to fibrocartilage to calcified cartilage and then finally bone. Some ligaments (and tendons) attach to the periosteum first, which then attaches to the bone. The site of ligament failure is a function of the load it experiences. Ligaments resist slow loading better than rapid loading. Therefore, rapid loading may produce an intraligamental lesion, whereas a slower pattern of loading will create injuries at or near the bone– ligament interface.
The ligaments of the knee. Because of the inherent instability of the joint, ligaments are necessary to prevent motion in all planes. They act as the primary stabilizers of the joint and are assisted by the muscles and other connective tissues.
Elastin is a protein that permits elastic recoiling to occur in a tissue. Some ligaments, such as the cruciate ligament of the knee, contain almost no elastin. Other ligaments, such as the ligamentum flavum of the spine, contain large amounts of elastin. shows that because it contains more collagen than elastin, the anterior cruciate ligament can resist tensile loads with little elongation. In this way, the anterior cruciate ligament serves the knee well as a stabilizing structure. On the other hand, the ligamentum flavum of the spine, being composed mostly of elastin and little collagen, can be stretched a great deal before breaking, but can only resist very weak tensile loading.
Ligaments function to limit joint motion and to guide the bones as they move. Ligaments therefore usually have a dual internal structure, such that they may stabilize the joint at either extreme of motion. Ligaments are most lax at midrange of joint motion. The capsule of a synovial joint is in fact a weak ligamentous structure. Disruption of a ligament can result in severe joint instability and increased frictional stresses to the articular surfaces of that joint. This will result in premature osteoarthritis. Conversely, a loss of normal capsular laxity from fibrosis following trauma will result in a severe restriction in joint motion (i.e., posttraumatic adhesive capsulitis of the shoulder).
Ligaments have very little vascularity; hence they heal poorly. However, they do have innervation, which may be useful to quantify the severity of a given ligamentous injury. When the structural integrity of a ligament has been completely compromised (grade III sprain), relatively little pain is produced on attempts to passively stretch the injured ligament. This is because no tension load can be created across a completely disrupted ligament. However, in a less severe partial tear (grade I sprain), severe and exquisite pain will be produced when tension is applied across the damaged structure. This paradoxical pain pattern (less pain equals a more severe sprain) can be a significant diagnostic clue obtained during the physical examination of a recently injured ligament. This also has dramatic import in defining a patient’s prognosis and determining a treatment plan.
Muscle
Skeletal muscle is a contractile tissue made up of fibers that contain specialized proteins (Figures and ). A loose connective tissue known as endomysium fills the space between these fibers. This tissue attaches to a stronger connective tissue that surrounds the muscle vesiculae, known as perimysium. Perimysium is in turn connected to the epimysium, which encases the entire muscle. This in turn is anchored to the fascial tissues of the nearby structures. Muscles therefore are composed of two elements: contractile tissues and inert, noncontractile tissues. The forces generated by the muscles are extrinsically applied to the muscle and will affect both types of tissue.
Muscles exist in many shapes and sizes. Some of these are shown in .
Muscles contain three different fiber types: I, IIa, and IIb. They are defined by the chemical machinery used to generate adenosine triphosphate (ATP). Genetic makeup, training, and neuromuscular disease can affect the composition of a given muscle with respect to fiber type. Characteristics of these various fiber types are shown in .
The mechanical response of stress and strain on the anterior longitudinal ligament and the ligamentum flavum. The anterior cruciate ligament, having more collagen than elastin, can handle a larger load but will only stretch a short amount before breaking. The ligamentum flava, having more elastin than collagen, cannot tolerate a very large load but can stretch a lot before breaking.
Muscles act to move body parts or to stabilize a joint. As dynamic stabilizers of joints, muscles serve to duplicate the static stabilizing action of ligaments. Muscle fibers are capable of shortening to about 50% of their original length. The tension developed by a contracted muscle can be either active or passive. Active tension is due to the contractile components, namely, actin and myosin. Passive tension results from elastic properties of the contractile tissues within the muscle.
The strength of the muscle is proportional to its cross-sectional area and mass. The force of contraction of a muscle is related to many factors, including the length of the fibers, the velocity of contraction, and the direction in which the fiber is moving at the time of its contraction. Types of muscle contraction include concentric or shortening, eccentric or lengthening, and isometric, in which the muscle does not change length. Muscles are characterized by their function; agonists are prime movers, antagonists resist the action of prime movers, and synergists support the function of the agonists. For example, in ankle dorsiflexion, the anterior tibialis is the agonist. The extensor hallucis longus and extensor digitorum longus muscles assist the tibialis anterior muscle and therefore are synergists. The gastrocnemius and soleus and plantar flexors of the toes are antagonists of the tibialis anterior.
Muscles are described in anatomy texts as having origins and insertions. It is very important to recognize that this is an arbitrary distinction. A muscle that is referred to as a hip flexor because it brings the thigh toward the torso can function just as well to bring the torso over the thigh. In order for muscles to function normally, they must be both strong and flexible.
With respect to innervation of muscles, except for the deepest layers of the vertebral muscles, the exact innervation of the limb and trunk muscles is similar between individuals, with some variability. Tables listing segmental innervation differ from text to text. Injuries to muscles are termed strains. Analogous to ligament injuries, they are classified by severity into three grades: grade I indicates minimal damage; grade II represents an intermediate amount of damage to the muscle structure; and grade III, complete disruption.
A microscopic view of muscle shows the repeated patterns of the sarcomeres and the fibrils.
Tendons
Tendons connect muscles to other structures (see ). Like ligaments, tendons are also composed of collagen, ground substance, and cells. The collagen of tendons is aligned in a very strict linear fashion and is always oriented in the line of the pull of the muscle. Tendons have been designed to transmit the force of the muscular contractile tissues to bone and other connective tissues, such as skin and ligaments, to which they are attached. Tendons are said to be able to withstand at least twice the maximum force that muscles can exert on them. The zone where the muscle blends into the tendinous tissues is called the musculotendinous junction. Muscle–tendon units represent tensile structures. As such, they may fail in the muscle, at the muscle–tendon junction, within the tendon, or at the tendon–bone insertion. Most commonly, however, failure occurs at the point of transition between two different materials (i.e., the musculotendinous junction). Some tendons are surrounded by a double-walled tubular covering, referred to as a tendon sheath or a peritendon (i.e., Achilles tendon or flexor tendons of the hand). This is lined with a synovial membrane. The sheath is used both to lubricate the tendon and to guide it toward the bony attachment. Tendon sheaths provide a pathway for the gliding movement of the tendon within the sheath. An inflamed tendon sheath can cause a locking or restricted movement, as in a trigger finger. Inflammation of the tendon structure is termed tendinitis.
The organization of skeletal muscle tissue.
Different types of muscle–fascicle arrangements.
Characteristics of skeletal muscle fibers based on their physical and metabolic properties.
ATP, adenosine triphosphate.
Synovium and Bursae
Synovial tissue lies in the inner aspect of synovial joints and bursal sacs. It has two functions: to produce lubricating fluids and to phagocytize (remove) foreign debris. Synovium is highly vascularized and innervated. As such, when traumatized or inflamed, synovial tissue will rapidly enlarge and produce significant pain.
Atendon.
The olecranon bursa is between the skin and the olecranon process at the elbow.
Bursal sacs serve to reduce friction. Therefore, they are located wherever there is need for movement between structures in close proximity. For example, the olecranon bursa lies between the olecranon process of the ulna and the skin overlying the posterior part of the elbow (see ). The subacromial bursa lies between the acromioclavicular arch above and the rotator cuff tendons below. Inflammation of synovial or bursal tissues due to trauma, inflammatory processes, or foreign materials is termed synovitis or bursitis.
Fascia
There are three kinds of fascial tissues: superficial, deep, and subserous. The fascia is composed of loose to dense connective tissue. Superficial fascia is under the skin; deep fascia is beneath the superficial and also envelops the head, trunk, and limbs. Subserous fascia surrounds organs in the thorax, abdomen, and pelvis.
Superficial fascia contains fat, blood vessels, and nerves. It is loose in consistency and very thin. It is attached to the undersurface of the skin.
Deep fascia is dense and tough and has two layers. It wraps around regions of the body and splits to envelop superficial muscles such as the sartorius and tensor fasciae latae. Periosteum, perimysium, and perichondrium are all elements of the deepest layer of the deep fascia. The deep fascia serves to interconnect the different muscle groups. By being continuous, it can provide tension at a distant site when pulled by a contracting muscle. Some muscles take their origin from the deep fascia. The fascia also separates groups of muscles with similar function, for example, the flexor and extensor groups of the leg. Because of the relative inelasticity of fascia, abnormally high pressure within a fascial compartment (i.e., due to injury or inflammation) can compromise the function of the nerves and blood vessels that course through that compartment. This may result in serious compromise of the tissues supplied by these nerves and vessels. Fascia may, as other tissues, experience an inflammatory reaction, fasciitis. This condition can be accompanied by moderate or even severe discomfort and scarring (fibrosis). Fibrosis can lead to stiffness and restricted movement.