Table of Contents
Cover
Title page
Copyright page
List of contributors
Preface
1 Hazardous responses of the solid Earth to a changing climate
Introduction
Climate change as a driver of geological and geomorphological hazards at glacial–interglacial transitions
Projected future climate changes and the potential for a geospheric response
Climate forcing of hazards in the geosphere
Conclusions
Acknowledgements
2 Projected future climate changes in the context of geological and geomorphological hazards
Introduction
Climate change research: informing mitigation and adaptation
Climate forcing of hazards in the geosphere
Conclusions
Acknowledgements
3 Climate change and collapsing volcanoes: evidence from Mount Etna, Sicily
Introduction
Lateral collapse at Mount Etna
Flank failure and watershed abandonment at Mount Etna
Cosmogenic 3He exposure dating of channel abandonment at Mount Etna
Results and interpretations
Conclusion
Acknowledgements
4 Melting ice and volcanic hazards in the twenty-first century
Introduction
What are hazards for ice- and snow-covered volcanoes, and where are they found?
Hazards for ice- and snow-covered volcanoes
How is ice thickness on volcanoes currently changing?
How has ice recession affected volcanic activity in the past?
How does the rate and extent of current ice melting compare with past changes?
How might hazards be affected by melting of ice and snow?
What are the likely effects of twenty-first century climate change on hazards at ice-covered volcanoes?
Was the 2010 eruption of Eyjafjallajökull triggered by climate change?
Gaps in our knowledge and targets for future research
Acknowledgements
5 Multiple effects of ice load changes and associated stress change on magmatic systems
Introduction
Effects of glacial unloading on deep magma generation
Influence on magma capture in the crust
Influence on shallow magma chambers
Discussion and conclusions
6 Response of faults to climate-driven changes in ice and water volumes at the surface of the Earth
Introduction
General model set-up and results
Case studies
Implications for other formerly glaciated mountain ranges and for regions currently experiencing ice-mass loss
Conclusions
Acknowledgements
7 Does the El-Niño – Southern Oscillation influence earthquake activity in the eastern tropical Pacific?
Introduction
ENSO and the seismicity of the East Pacific Rise
Origins and distribution of seismic activity on the East Pacific Rise
Statistical modelling of sea level and ENSO influence on earthquakes
Conclusion
Acknowledgements
8 Submarine mass failures as tsunami sources – their climate control
Introduction
Submarine mass failures
Landslide territories
SMFs, tsunamis and climate control
Conclusions
Acknowledgements
9 High-mountain slope failures and recent and future warm extreme events
Introduction
Case studies
Assessing changes in warm event frequencies based on RCM simulations
Discussion and conclusions
Acknowledgements
10 Impacts of recent and future climate change on natural hazards in the European Alps
Introduction
Aims and structure of this chapter
Climate and environment of the European Alps
Future climate patterns in the European Alps
Discussion
Implication for natural hazard and risk management
Conclusions and wider implications
11 Assessing the past and future stability of global gas hydrate reservoirs
Introduction
Gas hydrate structure
Where are gas hydrates found?
How much gas hydrate is there?
Formation and break down of gas hydrates
Hydrates and past climate changes
Future global gas hydrate hazards
Conclusions
Acknowledgements
12 Methane hydrate instability: a view from the Palaeogene
Introduction
The PETM and methane hydrates
Sedimentological evidence for Palaeogene mass movements
Magnitude of PETM carbon release
PETM climate sensitivity
Concluding remarks
Acknowledgements
Index
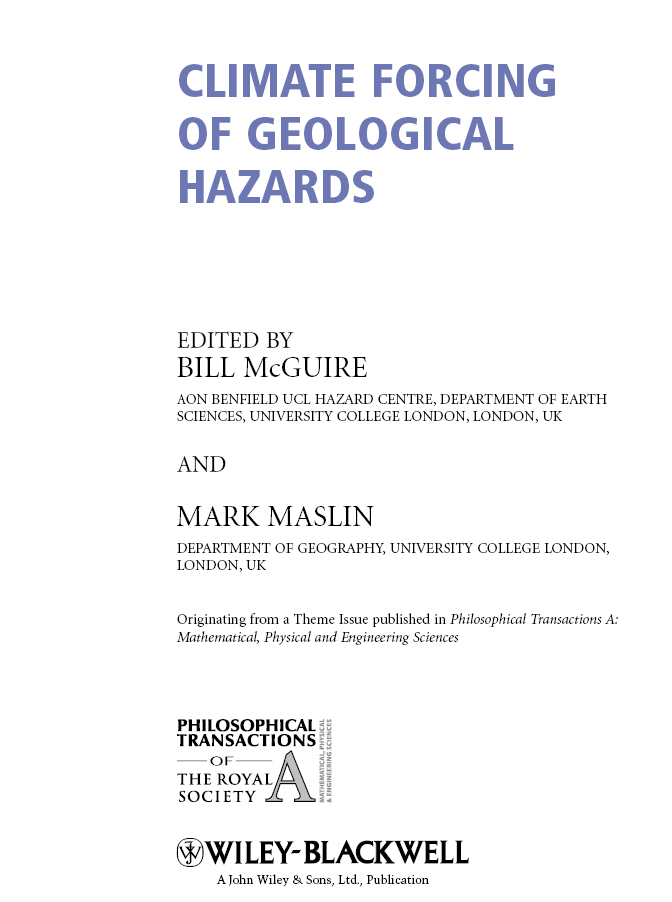
This edition first published 2013 © 2013 by The Royal Society and John Wiley & Sons, Ltd.
Wiley-Blackwell is an imprint of John Wiley & Sons, formed by the merger of Wiley’s global Scientific, Technical and Medical business with Blackwell Publishing.
Registered office: John Wiley & Sons, Ltd, The Atrium, Southern Gate, Chichester, West Sussex, PO19 8SQ, UK
Editorial offices: 9600 Garsington Road, Oxford, OX4 2DQ, UK
The Atrium, Southern Gate, Chichester, West Sussex, PO19 8SQ, UK
111 River Street, Hoboken, NJ 07030-5774, USA
For details of our global editorial offices, for customer services and for information about how to apply for permission to reuse the copyright material in this book please see our website at www.wiley.com/wiley-blackwell.
The right of the author to be identified as the author of this work has been asserted in accordance with the UK Copyright, Designs and Patents Act 1988.
All rights reserved. No part of this publication may be reproduced, stored in a retrieval system, or transmitted, in any form or by any means, electronic, mechanical, photocopying, recording or otherwise, except as permitted by the UK Copyright, Designs and Patents Act 1988, without the prior permission of the publisher.
Designations used by companies to distinguish their products are often claimed as trademarks. All brand names and product names used in this book are trade names, service marks, trademarks or registered trademarks of their respective owners. The publisher is not associated with any product or vendor mentioned in this book. This publication is designed to provide accurate and authoritative information in regard to the subject matter covered. It is sold on the understanding that the publisher is not engaged in rendering professional services. If professional advice or other expert assistance is required, the services of a competent professional should be sought.
Limit of Liability/Disclaimer of Warranty: While the publisher and author have used their best efforts in preparing this book, they make no representations or warranties with the respect to the accuracy or completeness of the contents of this book and specifically disclaim any implied warranties of merchantability or fitness for a particular purpose. It is sold on the understanding that the publisher is not engaged in rendering professional services and neither the publisher nor the author shall be liable for damages arising herefrom. If professional advice or other expert assistance is required, the services of a competent professional should be sought.
Library of Congress Cataloging-in-Publication Data
Climate forcing of geological hazards / edited by Bill McGuire and Mark Maslin.
p. cm.
“Originating from a theme issue published in Philosophical transactions A: mathematical, physical and enginering sciences”.
Includes bibliographical references and index.
ISBN 978-0-470-65865-9 (cloth)
1. Climatic changes. 2. Natural disasters. I. McGuire, Bill, 1954– II. Maslin, Mark. III. Philosophical transactions. Series A, Mathematical, physical, and engineering sciences.
QC903.C566 2013
363.34'1–dc23
2012030742
A catalogue record for this book is available from the British Library.
Wiley also publishes its books in a variety of electronic formats. Some content that appears in print may not be available in electronic books.
Cover image: Volcan Eyjafjallajökull in South Iceland. © iStockphoto.com/JochenScheffi
Cover design: Nicki Averill Design & Illustration
List of Contributors
Fabien Albino Nordic Volcanological Centre, Institute of Earth Sciences, University of Iceland, Reykjavik, Iceland
Simon Allen Climate and Environmental Physics, Physics Institute, University of Bern, Switzerland
Richard A. Betts Met Office Hadley Centre, Exeter, UK
Simon Day Aon Benfield UCL Hazard Centre, Department of Earth Sciences, University College London, London, UK
Kim Deeming School of Earth, Atmospheric and Environmental Sciences, University of Manchester, Manchester and Aon Benfield UCL Hazard Centre, Department of Earth Sciences, University College London, Gower Street, London, UK
Tom Dunkley Jones School of Geography, Earth and Environmental Sciences, University of Birmingham, Birmingham, UK
Rachel Flecker School of Geographical Sciences, University of Bristol, Bristol, UK
Serge Guillas Department of Statistical Science and Aon Benfield UCL Hazard Centre, University College London, London, UK
Andrea Hampel Institut für Geologie, Leibniz-Universität Hannover, Hannover, Germany
Stephan Harrison College of Life and Environmental Sciences, University of Exeter, Penryn, UK
Paul Harrop Tessella plc, Abingdon, Oxfordshire, UK
Ralf Hetzel Institut für Geologie und Paläontologie, Westfälische Wilhelms-Universität Münster, Germany
Andrew Hooper Delft University of Technology, Delft, The Netherlands
Christian Huggel Department of Geography, University of Zurich, Switzerland
Ruža F. Ivanovi
School of Geographical Sciences, University of Bristol, Bristol, UK
Jasper Knight School of Geography, Archaeology and Environmental Studies, University of the Witwatersrand, Johannesburg, South Africa
Margreth Keiler Geographical Institute, University of Bern, Bern, Switzerland
Felicity Liggins Met Office Hadley Centre, Exeter, UK
Björn Lund Department of Earth Sciences, Uppsala University, Sweden
Daniel J. Lunt School of Geographical Sciences, University of Bristol, Bristol, UK
Bill McGuire Aon Benfield UCL Hazard Centre, Department of Earth Sciences, University College London, London, UK
Georgios Maniatis Institut für Geologie, Leibniz-Universität Hannover, Hannover, Germany
Mark Maslin Department of Geography, University College London, London, UK
Matthew Owen Department of Geography, University College London, London, UK
Carolina Pagli School of Earth and Environment, University of Leeds, UK
Virginie Pinel ISTerre, IRD R219, CNRS, Université de Savoie, Le Bourget du Lac, France
Andrew Ridgwell Department of Geography, Bristol University, Bristol
Nadine Salzmann Department of Geography, University of Zurich, and Department of Geosciences, University of Fribourg, Switzerland
Peter Schmidt Department of Earth Sciences, Uppsala University, Sweden
Freysteinn Sigmundsson Nordic Volcanological Centre, Institute of Earth Sciences, University of Iceland, Reykjavik, Iceland
David R. Tappin British Geological Survey, Nottingham, UK
Hugh Tuffen Lancaster Environment Centre, Lancaster University, Lancaster, UK
Paul J. Valdes School of Geographical Sciences, University of Bristol, Bristol, UK
Preface
Since the Last Glacial Maximum, some 20,000 years ago, our world has experienced an extraordinary metamorphosis: flipping – in the blink of an eye, geologically speaking – from a frigid wasteland into the temperate world upon which our civilisation has grown and thrived. Over this period, a staggering 52 million cubic kilometres of water were redistributed about the planet, as the great continental ice sheets melted and previously depleted global sea levels rose 130 m to compensate. Rapid global warming, of around 6°C, resulted in atmospheric circulation patterns changing to accommodate broadly warmer, wetter conditions, leading to a modification of major wind trends and a rearrangement of climatic zones, but these were not the only consequences of our world’s dramatic post-glacial transformation. The solid Earth was involved too, as the lithosphere (the brittle, outer layer of our planet that comprises the crust and uppermost mantle) underwent major readjustments in response to the massive changes in water and ice load. Outcomes included major earthquakes in formerly ice-covered regions at high latitudes and a spectacular rise in the level of volcanic activity in Iceland.
The 12 chapters that make up this book together address the many and varied ways in which dramatic climate change, such as that which characterised post-glacial times, is able to ‘force’ a reaction from the solid Earth, or Geosphere. Of critical importance from a societal point of view, the prospects for anthropogenic climate change driving a hazardous response are also examined and evaluated. The book builds on presentations and dialogue at the Third Johnston–Lavis Colloquium held at University College London in September 2009. The meeting brought together delegates from the UK, Europe and the USA to address the issue of climate forcing of geological and geomorphological hazards, with a particular focus on examining possibilities for a geospheric response to anthropogenic climate change. The chapters that form this volume are a reflection of new research and critical reviews presented in sessions on: climates of the past and future; climate forcing of volcanism and volcanic activity; and climate as a driver of seismic, mass movement and tsunami hazards.
Two introductory papers set the scene. In the first, Bill McGuire summarizes evidence for periods of exceptional past climate change eliciting a dynamic response from the Earth’s lithosphere, involving enhanced levels of potentially hazardous geological and geomorphological activity. The response, McGuire notes, is expressed mainly through the triggering, adjustment or modulation of a range of crustal and surface processes that include gas-hydrate destabilisation, submarine and subaerial landslide formation, debris flow occurrence and glacial outburst flooding, and volcanic and seismic activity. Adopting a uniformitarian approach, and acknowledging potential differences in both rate and scale from the period of post-glacial warming, he goes on to examine potential influences of anthropogenic climate change in relation to an array of geological and geomorphological hazards across an assortment of environmental settings. In a second and complementary review paper, Felicity Liggins, and others, evaluate climate change projections from both global and regional climate models in the context of geological and geomorphological hazards. The authors observe that, in assessing potential for a geospheric response, it seems prudent to consider that regional levels of warming of 2°C are unavoidable, with high-end projections associated with unmitigated emissions potentially leading to a global average temperature rise in excess of 4°C, and far greater warming in some regions. Importantly, they note that significant uncertainties exist, not only in relation to climate projections, but also in regard to links between climate change and geospheric responses.
Between them, the following two chapters examine the ways and means whereby rapid climate change has, in the past, increased levels of volcanic activity and the destabilisation of volcanic edifices and promoted magma production, and look ahead to possible ramifications for volcanic landscapes of contemporary climate change. In Chapter 3, Kim Deeming and her co-authors explore the phenomenon of volcano lateral collapse (the large-scale failure and collapse of part of a volcano’s flank) in response to a changing climate. The authors present the results of a cosmic ray exposure dating campaign at Mount Etna in Sicily, which constrains the timing and nature of collapse of the Valle del Bove – a major volcanic landslide scar on the eastern flank of the volcano. The authors link pluvial (wet) conditions during the Early Holocene to the formation of a high-energy surface drainage system and to its truncation by a catastrophic lateral collapse event, about 7500 years ago, which opened the Valle del Bove. A possible mechanism is proposed whereby magma emplacement into a water-saturated edifice caused the thermal pressurization of pore-water, leading to a reduction in sliding resistance and subsequent large-scale slope failure. Deeming and her colleagues showcase the mechanism as one possible driver of future lateral collapse at ice-capped volcanoes and at those located in regions predicted to experience enhanced precipitation.
Following on from this, Hugh Tuffen provides a general evaluation of the impact of a changing climate on glaciated volcanoes – looking ahead to how the melting of ice caps on active volcanoes may influence volcanic hazards in the twenty-first century. In reviewing the evidence for current melting of ice increasing the frequency or size of future eruptions, he notes that much remains to be understood in relation to ice loss and increased eruptive activity. In particular, uncertainty surrounds the sensitivity of volcanoes to small changes in ice thickness and how rapidly volcanic systems respond to deglaciation. Nevertheless, Tuffen expects an increase in explosive eruptions at glaciated volcanoes that experience significant ice thinning, and a greater frequency of lateral collapse at glaciated stratovolcanoes in response to anthropogenic warming. On the positive side, deglaciation may ultimately reduce the threat from volcanic debris flows (lahars) and melt-water floods from volcanoes that currently support ice caps.
There is strong evidence for a lithospheric response to the rapidly changing post-glacial climate being elicited by load changes, either as a consequence of unloading at high latitudes and high altitudes due to ice-mass wastage, or as a result of the loading of ocean basins and continental margins in response to a ≥100 m rise in global sea level. In Chapter 5, Freysteinn Sigmundsson and his co-workers evaluate the influence of climate-driven ice loading and unloading on volcanism, focusing on Iceland and, in particular, the Vatnajökull Ice Cap. They note that ice wastage on Icelandic volcanoes reduces pressure at the surface and causes stress changes in magmatic systems. This in turn is capable of promoting an increase in the generation of magma in the uppermost mantle, raising the potential for the ‘capture’ of magma in the crust – as opposed to its eruption at the surface – and modifying the conditions required for the walls of a magma reservoir to fail. The authors demonstrate that, although pressure-release melting in the mantle may generate an amount of magma comparable with that arising from plate tectonic processes, at least part of this will never reach the surface. Perhaps somewhat surprisingly, Sigmundsson and his colleagues show that long-term ice wastage at Katla volcano may actually reduce the likelihood of eruption, because more magma is needed in the chamber to cause failure, compared with times when ice cover is greater.
Continuing the loading–unloading theme in Chapter 6, Andrea Hampel and her fellow researchers examine how active faults have responded to variations in ice and water volumes as a consequence of past climate change. Using numerical models, the authors demonstrate that climate-driven changes in ice and water volume are able to affect the slip evolution of both thrust and normal faults, with – in general – both the slip rate and the seismicity of a fault increasing with unloading and decreasing with loading. Adopting a case-study approach, Hampel and colleagues provide evidence for a widespread, post-glacial, seismic response on faults located beneath decaying ice sheets or glacial lakes. Looking ahead, the authors point to the implications of their results for ice-mass loss at high latitudes, and speculate that shrinkage of the Greenland and Antarctic ice sheets as a consequence of anthropogenic warming could result in a rise in the frequency of earthquakes in these regions.
In the next chapter, Serge Guillas and others provide a contemporary slant to loading and unloading effects by presenting the results of a statistical analysis of a putative correlation between recent variations in the El Niño–Southern Oscillation (ENSO) and the occurrence of earthquakes on the East Pacific Rise (EPR). The authors observe a significant (95% confidence level) positive influence of the Southern Oscillation Index (SOI) on seismicity, and propose that increased seismicity on the EPR arises due to the reduced sea levels in the eastern Pacific that precede El Niño events, which can be explained in terms of the reduction in ocean-bottom pressure over the EPR by a few kilopascals. Guillas and co-authors note that this provides an example of how variations in the atmosphere and hydrosphere can drive very small changes in environmental conditions which, in turn, are able to trigger a response from the solid Earth. Perhaps most significantly, they speculate that, in a warmer world, comparable and larger changes associated with ocean loading due to global sea level rise, or unloading associated with the passage of more intense storms, may trigger more significant earthquake activity at fault systems in the marine and coastal environments that are in a critical state.
Staying in the marine environment, Dave Tappin provides, in Chapter 8, a comprehensive review of the role of climate in promoting submarine mass failures (SMFs) that may source tsunamis. Tappin highlights the importance of climate in ‘preconditioning’ sediment so as to promote instability and failure, including its influence on sediment type, deposition rate and post-depositional modification. The author also notes that climate may play a role in triggering SMFs via earthquake or cyclic loading associated with tides or storm waves. Tappin makes the important point that, in the past, climate influence on SMFs appears to have been greatest at high latitudes and associated with glaciation–deglaciation cycles, which had a significant influence on sedimentation, preconditioning and triggering. In fact many of these current geohazards are due to the continued isostatic rebound as the land recovers from ice sheet loading more than 10,000 years ago. As a corollary, Tappin observes that, as the Earth warms, increased understanding of the influence of climate will help to underpin forecasting of tsunami-sourcing SMFs, in particular at high latitudes where climate change is occurring most rapidly.
The theme of slope destabilisation and failure, this time in a subaerial setting, is continued in the next chapter by Christian Huggel and his co-researchers, who examine recent large slope failures in the light of short-term, extreme warming events. Huggel and colleagues demonstrate a link between large slope failures in Alaska, New Zealand and the European Alps, and preceding, anomalously warm episodes. The authors present evidence supporting the view that triggering of large slope failures in temperature-sensitive high mountains is primarily a function of reduced slope strength due to increased production of meltwater from snow and ice, and rapid thaw processes. Looking ahead they expect more frequent episodes of extreme temperature to result in a rise in the number of large slope failures in elevated terrain and warn of potentially serious consequences for mountain communities.
In Chapter 10, Jasper Knight and others give a regional perspective on the slope failure and flood hazard in mountainous terrain, focusing on the influence of contemporary climate change on a broad spectrum of geomorphological hazards in the eastern European Alps, including landslides, rock falls, debris flows, avalanches and floods. In the context of the pan-continental 2003 heat wave and the 2005 central European floods, the authors demonstrate how physical processes and human activity are linked in climatically sensitive alpine regions that are prone to the effects of anthropogenic climate change. Importantly, Knight and colleagues note that, although the European Alps, alongside other glaciated mountain ranges, are being disproportionately impacted upon by climate change, this is further exacerbated by regional factors including local climatology and long-term decay of glaciers and permafrost. The authors conclude that future climate changes are likely to drive rises in the incidence of mountain hazards and, consequently, increase their impact on Alpine communities.
The two concluding chapters centre on gas hydrates (or clathrates) and their sensitivity to a rapidly changing climate. In both marine and continental settings, gas-hydrate deposits have long captured interest, both in relation to their potential role in past episodes of sudden warming, such as during the Paleocene–Eocene Thermal Maximum (PETM), some 55 million years ago, and in the context of future anthropogenic warming. In the first, Mark Maslin and his fellow researchers review the current state of the science as it relates to the hazard potential of gas hydrates. Maslin and colleagues note that gas hydrates may present a serious threat as the world warms, primarily through the release into the atmosphere of large quantities of methane, which is an extremely effective greenhouse gas, resulting in accelerated global warming. In addition, they observe that the explosive release of methane from gas hydrates may also promote submarine slope failure and the consequent generation of potentially destructive tsunamis. The authors also stress, however, that, although the destabilisation of gas hydrates in permafrost terrains can be robustly linked to projected temperature increases at high latitudes, it remains to be determined whether or not future ocean warming will lead to significant methane release from marine hydrates.
In a second paper, and the last chapter of the volume, Tom Dunkley Jones and others look back to the PETM, the most prominent, transient global warming event during the Cenozoic, in order to evaluate the effects of the rapid release of thousands of gigatonnes of greenhouse gases on the planet’s climate, ocean-atmosphere chemistry and biota, for which the PETM provides perhaps the best available analogue. Dunkley Jones and his co-workers support the view that, although gas hydrate release was probably not responsible for an initial, rapid, CO2-driven warming, the as yet unknown event responsible for this subsequently triggered the large-scale dissociation of gas hydrates, which contributed to further warming as a positive feedback mechanism. As the authors note, this somewhat equivocal situation ensures that the question of what role – if any – gas hydrates may play in future anthropogenic warming, remains to be answered.
We feel that this book provides a valuable new insight into how climate change may force geological and geomorphological phenomena, ultimately increasing the risk of natural hazards in a warmer world. Taken together, the chapters build a panorama of a field of research that is only now becoming recognized as important in the context of the likely impacts and implications of anthropogenic climate change. We are keen for this volume to provide a marker that reinforces the idea that anthropogenic climate change does not simply involve the atmosphere and hydrosphere, but can also elicit a response from the Earth beneath our feet. In this regard we are hopeful that it will encourage further research into those mechanisms by which climate change may drive potentially hazardous geological and geomorphological activity, and into the future ramifications for society and economy.
Bill McGuire and Mark Maslin
London, UK
This book was originally published as an issue of the Philosophical Transactions A: Mathematical, Physical and Engineering Sciences (volume 368, issue 1919) but has been materially changed and updated.
1
Hazardous Responses of the Solid Earth to a Changing Climate
Bill McGuire
Aon Benfield UCL Hazard Centre, Department of Earth Sciences, University College London, UK
Summary
Periods of exceptional climate change in Earth’s history are associated with a dynamic response from the geosphere, involving enhanced levels of potentially hazardous geological and geomorphological activity. The response is expressed through the adjustment, modulation or triggering of a broad range of surface and crustal phenomena, including volcanic and seismic activity, submarine and subaerial landslides, tsunamis and landslide ‘splash’ waves, glacial outburst and rock-dam failure floods, debris flows and gas-hydrate destabilisation. In relation to anthropogenic climate change, modelling studies and projection of current trends point towards increased risk in relation to a spectrum of geological and geomorphological hazards in a warmer world, whereas observations suggest that the ongoing rise in global average temperatures may already be eliciting a hazardous response from the geosphere. Here, the potential influences of anthropogenic warming are reviewed in relation to an array of geological and geomorphological hazards across a range of environmental settings. A programme of focused research is advocated in order to: (1) better understand those mechanisms by which contemporary climate change may drive hazardous geological and geomorphological activity; (2) delineate those parts of the world that are most susceptible; and (3) provide a more robust appreciation of potential impacts for society and infrastructure.
Introduction
Concern over anthropogenic climate change driving hazardous geological and geomorphological activity is justified on the basis of four lines of evidence: (1) periods of exceptional climate change in Earth’s history are associated with a dynamic response from the geosphere; (2) small changes in environmental conditions provide a means whereby physical phenomena involving the atmosphere and hydrosphere can elicit a reaction from the Earth’s crust and sometimes at deeper levels; (3) modelling studies and projection of current trends point towards increased risk in relation to a range of geological and geomorphological hazards in a warmer world; and (4) observations suggest that the ongoing rise in global average temperatures may already be eliciting a hazardous response from the geosphere.
A link between past climate change and enhanced levels of potentially hazardous geological and geomorphological activity is well established, with supporting evidence coming mostly, although not exclusively, from the period following the end of the Last Glacial Maximum (LGM) around 20 ka BP (20 thousands of years before present). During the latest Pleistocene and the Holocene, the atmosphere and hydrosphere underwent dramatic transformations. Rapid planetary warming promoted a major reorganisation of the global water budget as continental ice sheets melted to replenish depleted ocean volumes, resulting in a cumulative sea-level rise of about 130 m. Contemporaneously, atmospheric circulation patterns changed to accommodate broadly warmer, wetter conditions, leading to modification of major wind trends and a rearrangement of climatic zones.
The nature of the geospheric response to transitions from glacial to interglacial periods provides the context for evaluating the potential of current greenhouse gas (GHG)-related warming to influence the frequency and incidence of geological and geomorphological hazards. Critically, however, differences in the timescale, degree and rate of contemporary environmental change may result in a different hazardous response. The key question, therefore, is: to what extent does the post-glacial period provide an analogue for climate-change driven hazards in the twenty-first century and beyond.
Climate Change as a Driver of Geological and Geomorphological Hazards at Glacial–Interglacial Transitions
At the broadest of scales, modification of the global pattern of stress and strain, due to a major redistribution of planetary water, may influence geological and geomorphological activity at times of glacial–interglacial transition (Matthews, 1969; Podolskiy, 2008). As noted in Liggins et al. (2010), however, a more targeted geospheric response to planetary warming and hydrological adjustment during these times is associated with ice-mass loss, rapid sea-level rise and greater availability of liquid water, in the form of either ice melt or increased precipitation levels. These environmental transformations in turn drive load pressure changes and increases in pore-water pressure which, together, act to promote hazardous geological and geomorphological activity. Notably, variations in ice and water load have been linked to fault rupture (Hampel et al., 2007, 2010), magma production and eruption (McNutt & Beavan, 1987; McNutt, 1999; Pagli & Sigmundsson, 2008; Sigmundsson et al., 2010), and submarine mass movements (Lee, 2009; Tappin, 2010). Elevated pore-water pressures are routinely implicated in the formation of subaerial and marine landslides (Pratt et al., 2002; Tappin 2010).
The geospheric response to such changes in environmental conditions at times of glacial–interglacial transition is expressed through the adjustment, modulation or triggering of a wide range of surface and crustal phenomena, including volcanic (e.g. Chappel, 1975; Kennett & Thunell, 1975; Rampino et al., 1979; Hall, 1982; Wallmann et al., 1988; Nakada & Yokose, 1992; Sigvaldason et al., 1992; Jull & McKenzie, 1996; McGuire et al., 1997; Zielinksi et al., 1997; Glazner et al., 1999; Maclennan et al., 2002; Bay et al., 2004; Jellinek et al 2004; Nowell et al., 2006; Licciardi et al., 2007; Bigg et al., 2008; Carrivick et al., 2009a; Huybers & Langmuir, 2009) and seismic (e.g. Anderson, 1974; Davenport et al., 1989; Costain & Bollinger, 1996; Luttrell & Sandwell, 2010; Wu et al., 1999; Wu & Johnston, 2000; Hetzel & Hampel, 2005) activity, marine (e.g. Maslin et al., 1998, 2004; Day et al., 1999, 2000; Masson et al., 2002; Keating & McGuire, 2004; McMurtry et al., 2004; Vanneste et al., 2006; Quidelleur et al., 2008; Lee, 2009; Tappin, 2010) and subaerial (Lateltin et al., 1997; Friele & Clague, 2004; Capra, 2006) landslides, tsunamis (McMurtry et al., 2004; Lee, 2009) and landslide ‘splash’ waves (Hermanns et al., 2004), glacial outburst (Alho et al., 2005) and rock-dam failure (Hermanns et al., 2004), floods, debris flows (Keefer et al., 2000) and gas-hydrate destabilisation (e.g. Henriet & Mienert, 1998; Maslin et al., 2004; Beget & Addison 2007; Grozic, 2009).
The degree to which comparable responses to projected future climate changes could modify the risk of geological and geomorphological hazards is likely to be significantly dependent on the scale and rate of future climate change. The scale of changes in key environmental conditions in post-glacial times was considerable, with the rapid loss of continental ice sheets after the LGM, leading to cumulative load-pressure reductions on the crust of a few tens of megapascals, and sea levels in excess of 100 m higher increasing the total load on the crust by approximately 1 MPa. Rates of change were also dramatic, with annual vertical mass wastage of between 10 and 50 m (corresponding to a load reduction of 10–50 kPa) reported for the Wisconsin Laurentide Ice Sheet (Andrews, 1973). The rate of global eustatic sea level rise may have approached 5 m a century at times, with annual rates of more than 45 mm (Blanchon & Shaw, 1995), resulting in an annual load-pressure increase on the crust of about 1 kPa. Given the scale of absolute changes and the very high rates involved, it is unsurprising that imposition of such stresses within the crustal domain elicited a significant geological and geomorphological response. Although the post-LGM climate of the latest Pleistocene and the Holocene was characterised by considerable variability (Mayewski et al., 2004), the transition from ‘ice-world’ to ‘water-world’ broadly altered the moisture balance in favour of a far greater incidence of warm and wet conditions, e.g. during the African Humid Period from about 14,800 to 5500 years BP (Hély et al., 2009) and during the Early Holocene across much of the Mediterranean (Frisia et al., 2006). Higher levels of precipitation raised the potential for higher pore-water pressures in unstable volumes of rock and debris, e.g. promoting landslide formation. Pratt et al. (2002), speculate that enhanced monsoon rainfall during the Early Holocene raised pore-water pressures in the Nepal Himalayas, resulting in an increase in landslide frequency. Similarly, Capra (2006) invokes more humid Holocene conditions to explain an apparent increase in the incidence of lateral collapse of volcanic edifices. In relation to the formation of submarine landslides in the post-glacial period, a range of potential environmental triggers are proposed, most notably elevated levels of seismicity associated with isostatic rebound of previously ice-covered crust, or ocean-loading due to rapid sea-level rise, but also elevated sediment pore pressures and gas hydrate destabilisation (see Lee, 2009 for more comprehensive discussion of these factors).
Projected Future Climate Changes and the Potential for a Geospheric Response
Since the LGM, about 20 ka BP, global average temperatures have risen by around 6°C, with a rise of close to 0.8°C occurring in the last 100 years (Figure 1.1). Without a major change in energy policy, GHG emissions are projected to rise substantially, increasing the global mean temperature by between 1.6°C and 6.9°C relative to pre-industrial times, driving long-term rises in temperature and global sea level and possible changes to the Atlantic Meridional Overturning Circulation (MOC) (IPCC 2007) (Figure 1.2). As noted by Liggins et al. (2010), physical inertia in the climate system ensures that the full effect of past anthropogenic forcing remains to be realised. Considering that global GHG emissions are still on an upward trend, and with no binding agreement in place to reduce this, it is highly likely that warming will result in regional temperature rises of at least 2°C above the pre-industrial period. Under the high-end Intergovernmental Panel on Climate Change (IPCC) SRES (Special Report on Emissions Scenarios) emissions scenario (A1F1), Betts et al. (2011) show that global average temperatures are likely to reach 4°C relative to pre-industrial times by the 2070s, and perhaps as early as 2060. Under the lowest of the main emissions scenarios (B1), the central estimate of warming is projected to be 2.3°C relative to the pre-industrial period. These projections indicate that the current episode of GHG-driven warming is exceptional. As observed in the IPCC AR4 (IPCC 2007), if temperatures rise about 5°C by 2100, the Earth will have experienced approximately the same amount of warming in a few centuries as it did over several thousand years after the LGM. This rate of warming is not matched by any comparable global average temperature rise in the last 50 million years. Furthermore, high latitudes, where most residual ice now resides, are expected to warm even more rapidly. Christensen et al. (2007), for example, propose that under the A1B scenario the Arctic (north of 60° N) could warm by between 2.8°C and 7.8°C by 2080–2099 (relative to 1980–1999). ‘High-end’ projections under the A2 scenario suggest that surface temperatures across much of the Arctic could increase by 15°C by the 2090s (Sanderson et al., 2011).
Global temperature rises are driving increases in ocean volume due to thermal expansion of seawater and via melting of glaciers, ice caps, and the Greenland and West Antarctic ice sheets. Dependent on the scenario, annual thermosteric sea-level rise by 2100 could lie between 1.9 ± 1.0 (B1 scenario) and 3.8 ± 1.3 (A2) (Meehl et al., 2007). Total global mean sea-level rise by the end of the century is projected in the IPCC AR4 (IPCC 2007) to be between 0.18 and 0.59 m. Even the high end of these projections may, however, be an underestimate. Rahmstorf (2007), for example, argues for a rise of 0.5–1.4 m by the end of the century, whereas Pfeffer et al. (2008) estimate an upper bound of 2 m by 2100.
Projected changes in other climate quantities are also relevant in relation to influencing potentially hazardous crustal and surface processes, most notably variations in patterns of precipitation. Under the A1B scenario, for example, the Arctic (north of 60° N) is projected to see a 28% increase in precipitation by 2080–2099 relative to 1980–1999 (Christensen et al., 2007), and a similar rise is expected for Alaska and Kamchatka (Liggins et al., 2010). Ocean warming may also be important, and while this is projected to progress more slowly than over land masses (Meehl et al., 2007), it is expected to be greatest at high latitudes, where it may play a role in accelerating ice wastage and in contributing towards gas hydrate disassociation.
Projected rising temperatures and sea levels, and changes in precipitation, are capable of initiating load changes and elevated pore-water pressures that exceed levels that have been shown to drive a range of geological and geomorphological processes that have hazardous potential. Small ice masses are already experiencing serious wastage, with surging and thinning of some glaciers resulting in vertical mass reduction of tens to hundreds of metres (Doser et al., 2007), leading to load pressure declines on basement rocks of ≥0.5 MPa (Sauber et al., 2000). Comparable load pressure falls may be expected in relation to the Greenland and West Antarctic ice sheets if increased melting accelerates ice loss and glaciers surge and thin. The projected 0.18- to 0.59-m rise in sea level by the end of the century (IPCC 2007) would result in an increased load on the crust of 1.8–5.9 kPa. For a rise of up to 1.4 m (Rahmstorf, 2007), the load change rises to 14 kPa, and to 20 kPa for the upper bound 2.0 m rise of Pfeffer et al. (2008). Recently (1993–2003), annual sea level rise has been on the order of 2.4–3.8 mm/year (IPCC 2007), which translates broadly to an increased load pressure of 0.1 kPa every 3 years.
Although the load pressure changes associated with GHG-driven ice wastage and sea-level rise are generally small, in terms of both absolute values and rates, increasing evidence supports the view that they may be sufficient to trigger a geospheric response. Mounting evidence makes a convincing case for the modulation or triggering of seismic, volcanic and landslide activity as a consequence of small changes in environmental parameters such as solid Earth and ocean tides, and atmospheric temperature and pressure, as well as in response to specific geophysical events such as typhoons or torrential precipitation (Table 1.1).
Table 1.1 Examples of environmental drivers of seismicity, landslide slip and eruptive activity described in the literature, together with associated driving pressures
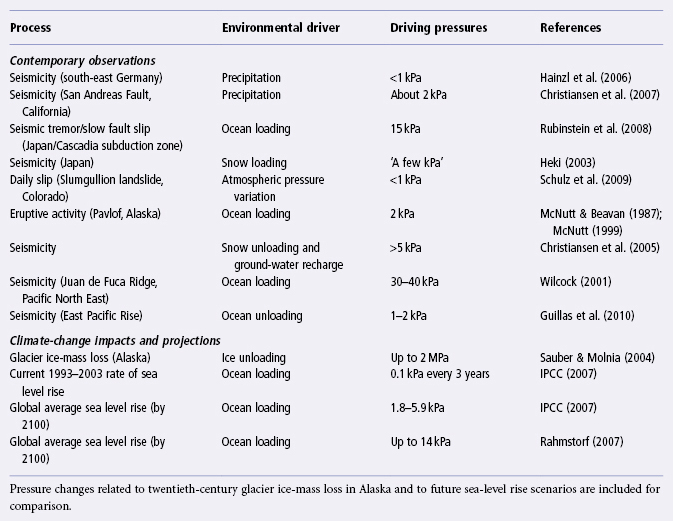
Based on observations of seismicity from south-east Germany, Hainzl et al. (2006) demonstrate that the crust can sometimes be so close to failure that even tiny (<1 kPa) pore-pressure variations associated with precipitation can trigger earthquakes in the top few kilometres. Christiansen et al. (2007) propose that modulation of seismicity on a creeping section of the San Andreas Fault in the vicinity of Parkfield is linked to the hydrological cycle. The authors suggest that fracturing of critically stressed rocks occurs either as a consequence of pore-pressure diffusion or crustal loading/unloading, and note that hydrologically induced stress perturbations of about 2 kPa may be sufficient to trigger earthquakes on the fault. In volcanic settings, Mastin (1994) relates the violent venting of volcanic gases at Mount St Helen’s between 1989 and 1991 to slope instability or accelerated growth of cooling fractures within the lava dome after rainstorms, whereas Matthews et al. (2002) link episodes of intense tropical rainfall with collapses of the Soufriere Hills’ lava dome on Montserrat (Caribbean).
Liu et al. (2009) show that slow earthquakes in eastern Taiwan are triggered by stress changes of approximately 2 kPa on faults at depth, associated with atmospheric pressure falls caused by passing tropical cyclones. Rubinstein et al. (2008) have been able to correlate episodes of slow fault slip and accompanying seismic tremor at subduction zones in Cascadia (Pacific North West) and Japan with the rise and fall of ocean tides, which involve peak-to-peak load pressure changes (for Cascadia) of 15 kPa. Heki (2003) demonstrates that snow load seasonally influences the seismicity of Japan through increasing compression on active faults and reducing the Coulomb failure stress by a few kilopascals. Schulz et al. (2009) show that diurnal tidal variations in atmospheric pressure amounting to <1 kPa modulate daily slip on the Slumgullion landslide in south-west Colorado. For volcanoes, Earth tides (Johnston & Mauk, 1972; Hamilton, 1973; Sparks, 1981) and other changing external factors, such as barometric pressure (Neuberg, 2000) or ocean loading (McNutt & Beavan, 1987; McNutt, 1999), have been proposed as having roles in forcing or modulating activity. McNutt and Beavan (1987) and McNutt (1999), for example, suggest that eruptions of the Pavlof (Alaska) volcano, from the early 1970s to the late 1990s, were modulated by ocean loading involving yearly, non-tidal, variations in local sea level as small as 20 cm, which translates to a load pressure change on the crust of 2 kPa. On a geographically broader scale, Bettinelli et al. (2008) explain seasonal variations in the seismicity of the Himalayas in terms of changes in surface hydrology, whereas Christiansen et al. (2005) link shallow (<3 km) seasonal seismicity at large calderas and stratovolcanoes across the western USA with stress changes of >5 kPa associated with snow unloading and ground-water recharge. Guillas et al. (2010) argue for reduced sea level in the eastern Pacific before the development of El Niño conditions, and approximating to a 1- to 2-kPa sea-bed load reduction, triggering increased levels of seismicity on the East Pacific Rise. At the global level, Mason et al. (2004) present evidence from the last 300 years in support of a seasonal signal in volcanic activity. This they attribute to fluctuations across a range of environmental conditions associated with the deformation of the Earth in response to the annual hydrological cycle, including reduced sea levels, millimetre-scale motion of the Earth’s crust and falls in regional atmospheric pressure. Although far from established, Podolskiy (2008) makes a case for an increase in global seismicity in recent decades, citing climate change as one potential driver.
Climate Forcing of Hazards in the Geosphere
In light of the above, the potential is addressed for enhanced responses to changing environmental conditions so as to increase the risk of geological and geomorphological hazards in a GHG-warmed world. In the context of rising atmospheric and ocean temperatures, ice-mass wastage and changing patterns of precipitation, possible implications are examined for high-latitude regions, ocean basins and margins, mountainous terrain and volcanic landscapes (Table 1.2 and Figure 1.3).
Table 1.2 Potential geological and geomorphological hazards in the context of projected future climate changes
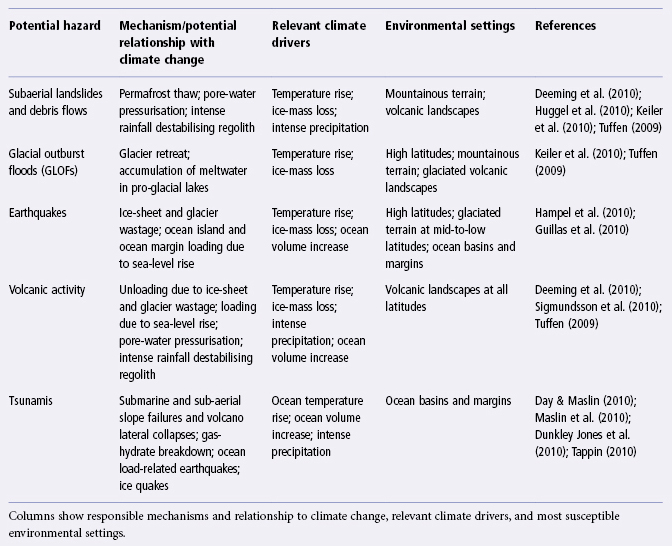
High Latitude Regions